The Dialectical Biologist (Richard Levins, Richard Lewontin)
The Dialectical Biologist | |
---|---|
Author | Richard Levins, Richard Lewontin |
First published | 1985 |
Type | Book |
Source | Ebin |
Preface
This book has come into existence for both theoretical and practical reasons. Despite the extraordinary successes of mechanistic reductionist molecular biology, there has been a growing discontent in the last twenty years with simple Cartesian reductionism as the universal way to truth. In psychology and anthropology, and especially in ecology, evolution, neurobiology, and developmental biology, where the Cartesian program has failed to give satisfaction, we hear more and more calls for an alternative epistemological stance. Holistic, structuralist, hierarchical, and systems theories are all offered as alternative modes of explaining the world, as ways out of the cul-de-sacs into which reductionism has led us. Yet all the while there has been another active and productive intellectual tradition, the dialectical, which is just now becoming widely acknowledged.
Ignored and suppressed for political reasons, in no small part because of the tyrannical application of a mechanical and sterile Stalinist diamat, the term dialectical has had only negative connotations for most serious intellectuals, even those of the left. Noam Chomsky once remarked to one of us, who accused him in a conversation of being insufficiently dialectical, that he despised the term and that in its best sense dialectics was only another way of saying “thinking correctly.” Now dialectics has once again become acceptable, even trendy, among intellectuals, as ancient political battles have receded into distant memory. In psychology, anthropology, and sociology, dialectical schools have emerged that trace their origins to Hegel.
In biology a school of dialectical analysis has announced itself as flowing from Marx rather than directly from Hegel. Its manifesto, issued at the Bressanone Conference in 1981 by the Dialectics of Biology Group, began, “A strange fate has overcome traditional Western philosophy of mind.” The Bressanone Conference did show the power of dialectical analysis as a critique of the current state of biological theory, although it left for the future the constructive application of a dialectical viewpoint to particular problems and, indeed, an explicit statement of what the dialectical method comprises.
As biologists who have been working self-consciously in a dialectical mode for many years, we felt a need to illustrate the strength of the dialectical view in biology in the hope that others would find a compelling case for their own intellectual reorientation. The essays in this book are the result of a long-standing intellectual and political comradeship. It began at the University of Rochester, where we worked together on theoretical population genetics and took opposite views on the desirability of mixing mental and physical labor (a matter on which we now agree). Later, working together at the University of Chicago and now at Harvard, in Science for Viet Nam and Science for the People, we have had more or less serious disagreements on intellectual and political tactics and strategy. But all the while, both singly and in collaboration, we have worked in a dialectical mode. Each of us separately has published a book that is dialectical in its explication, in the formulation of its problematic, and in the analysis of solutions (Richard Levins, Evolution in Changing Environments [Princeton University Press, 1968]; Richard Lewontin, The Genetic Basis of Evolutionary Change [Columbia University Press, 1974]). We believe that the considerable impact of these books, the one in ecology and the other in evolutionary biology, is a confirmation of the power of dialectical analysis. Both separately and together we have published scores of essays, applying the dialectical method, sometimes explicitly, sometimes implicitly, to scientific and political issues and to the relation of one to the other. Indeed, it is a sign of the Marxist dialectic with which we align ourselves that scientific and political questions are inextricably interconnected— dialectically related.
This book, then, is a collection of essays written at various times for various purposes and should be treated by the reader accordingly. Except for their grouping under general categories, the chapters do not have an ordered relation to one another. Material from some essays is recapitulated in others. The book does not follow a single logical development from first page to last but rather is meant to be a sampler of a mode of thought. That is why we have called it The Dialectical Biologist rather than Dialectical Biology, which would announce a single coherent project that we do not intend.
The particular essays we have chosen reflect a purely practical concern. Over the years much of what we have written has appeared in languages other than English and in publications not usually seen by biologists. We have repeatedly sent out photocopies of worn manuscripts, either in response to a request by someone who has heard a rumor of a certain essay or in an attempt to explicate a position. It seemed only sensible to collect these hard-to-find essays in one place, especially since they often represent the best expression of our point of view. We have taken the opportunity to do some editing. For the most part the changes are trivial, but in a few cases we have added some fresh material or inserted paragraphs from other essays to illuminate the argument. In one case, we have eliminated a large chunk of irrelevant didactic material.
After collecting these essays, we were dissatisfied. The assembled work illustrated the dialectical method, but it did not explain what dialectics is. Since the book is designed to be read by dissatisfied Cartesians, ought we not explicitly state our world view? Except for a sketch of it in “The Problem of Lysenkoism,” we nowhere touched on the subject. We then set about to write a chapter on dialectics—only to discover that in twenty-five years of collaboration we had never discussed our views systematically! The final chapter in this book is an attempt to make explicit what had been implicit in our understanding. It is only a first attempt. Like everything else, it will develop in the future as a consequence of its own contradictions.
We would like to express our gratitude to Michael Bradie, whose severe criticism improved the last chapter. We are immensely grateful, too, to Becky Jones, who helped make manageable order from a chaos of manuscripts, revisions, and additions.
ONE On Evolution
1 Evolution as Theory and Ideology
This chapter was first published as “Evoluzione” in Enciclopedia Einaudi, vol. 3, edited by Giulio Einaudi (Turin, Italy, 1977). A long section on the principles of evolutionary genetic change has been omitted here. The present text is the English original, which was translated into Italian for the encyclopedia.
The ideology of evolution
Although the concept of evolution has become firmly identified with organic evolution, the history of living organisms on earth, the theory of the evolution of life is only a special case of a more general world view that can be characterized as “evolutionism.” The ideology of evolutionism, which has developed in the last two hundred years, has permeated all the natural and social sciences, including anthropology, biology, cosmology, linguistics, sociology, and thermodynamics. It is a world view that encompasses the hierarchically related concepts of change, order, direction, progress, and perfectability, although not all theories of evolutionary processes include every successive step in the hierarchy of concepts. Theories of the evolution of the inorganic world, like cosmology and thermodynamics, generally include only change and order, while biological and sociological theories add the ideas of progress and even perfectability as elaborations of their theoretical structure.
Change
All evolutionary theories, whether of physical, biological, or social phenomena, are theories of change. The present state of a system is seen as different from its past states, and its future states are predicted to again differ from the present. But the simple assertion that past, present, and future differ from one another is not in itself an evolutionary world view. Before the widespread acceptance of evolutionary ideas in the nineteenth century, it was recognized that changes occur in natural and social systems, but these changes were regarded as exceptional alterations in a normally stable and static universe. The myth of the Noachian flood, by which God intervened to destroy the living world, only to repopulate it again from a handful of living beings especially preserved for that purpose, was the prototype of a general, nonevolutionary theory of change. The world had been specially created in both its natural and social form by the will of God, and the organization of the world was a manifestation of that divine will. On occasion the state of the world underwent an alteration, but such a change was abnormal, the result of divine intervention in an otherwise unchanging universe. The increasingly frequent discovery of fossils in the eighteenth and nineteenth centuries made it apparent that new forms of life had appeared at various epochs and that old forms had died out.
William Buckland’s (1836) response to these discoveries was characteristic: “In the course of our enquiry, we have found abundant proofs, both of the Beginning and the End of several successive systems of animal and vegetable life; each compelling us to refer its origin to the direct agency of Creative Interference; ‘We conceive it undeniable that we see, in the transition from an Earth peopled by one set of animals to the same Earth swarming with entirely new forms of organic life, a distinct manifestation of creative power transcending the operation of known laws of nature.’ ” The Noachian flood was generalized to a succession of floods in the theory of diluvianism, which was in turn part of the general theory called catastrophism, which included both floods and inundations by lava from periodic volcanic activity. In the domain of social organization, it was assumed that classes were fixed in their relations by divine will but that occasional changes in the social status of individuals could occur as the result of the withdrawal or conferral of grace either by God or his earthly representatives. Charles I ruled dei gratia, but as Oliver Cromwell observed, God’s grace was removed from him, as evidenced by his severed head.
There is no fundamental difference between a theory that the world was populated once by an act of special creation and one that postulates several such episodes. All theories of change by the occasional intervention of a higher power or extraordinary force in an otherwise static universe stand in direct opposition to the evolutionary world view, which sees change as the regular and characteristic feature of natural and social systems. In this uniformitarian view, the only unchanging features of the universe are the laws of change themselves. Uniformitarianism was first introduced into geology by James Hutton in 1785 and expanded by Sir Charles Lyell in his Principles of Geology (1830). According to the uniformitarian view, the geological processes of mountain building and erosion, which are responsible for the present features of the earth, have been at work ever since water in appreciable quantities has been present, and will continue to operate throughout the history of the earth with the same geotectonic consequences.
The theory of organic evolution assumes that the processes of mutation, recombination, and natural selection have been the driving forces since the beginning of life, even before its organization into cells, and that these forces will continue as a characteristic feature of living organisms until the extinction of the living world. It is assumed that life in other parts of the cosmos will exhibit these same dynamic features. A commitment to the evolutionary world view is a commitment to a belief in the instability and constant motion of systems in the past, present, and future; such motion is assumed to be their essential characteristic. In the eighteenth century this belief was expressed for the nascent bourgeois revolution by Diderot: “Tout change, tout passe, il n’y a que le tout qui reste” (everything changes, all things pass, only the totality remains) [1830] 1951, p. 56). In the nineteenth century Engels expressed the socialist revolutionary ideology: “Motion in the most general sense, conceived as the mode of existence, the inherent attribute, of matter, comprehends all changes and processes occurring in the universe, from mere change of place right up to thinking” ([1880] 1934, p. 69).
The growth in the ideology of change as an essential feature of natural systems was the necessary outcome of that slow but profound alteration in European social relations that we call the bourgeois revolution. The replacement of hereditary holders of power by those whose power derived from their entrepreneurial activities demanded an alteration in legitimating ideology from one of natural stasis and stability to one of unceasing change. The breaking down of the last vestiges of feudal society, in which peasant and lord alike were tied to the land; the ascendancy of merchants, financiers, and manufacturers; the growing power in France of the noblesse de la robe in parallel to the old noblesse de l’epee—all were in contradiction with a world view that saw changes in state as only occasional and unusual, the result of irregular reallocations of grace. Reciprocally, a world view that made change an essential feature of natural systems was inconceivable in a social world of fixed hereditary relations. Human beings see the natural world as a reflection of the social organization that is the dominant reality of their lives. An evolutionary world view, being a theory of the naturalness of change, is really congenial only in a revolutionizing society.
Order
Although change is a necessary feature of evolutionary ideology, it has not seemed sufficient to most evolutionary theorists. If a deck of cards is shuffled over and over, the sequence of cards changes continually, yet in some sense nothing is happening. One random sequence of cards is much like another, and successive states of the deck cannot be described except by enumerating the cards. For Bergson and Whitehead, for example, no evolution is occurring because there are only successive states of chaos, while an evolutionary process must give rise to new states of organization. In Science and the Modern World, Whitehead wrote: “Evolution, on the materialistic theory, is reduced to the role of being another word for the description of changes of the external relations between portions of matter. There is nothing to evolve, because one set of external relations is as good as any other set of external relations. There can merely be change, purposeless and unprogressive” ([1925] 1960, p. 157).
Nearly all evolutionary theories attempt to describe the outcome of the evolutionary process in terms of an ordered scale of states rather than simply as an exhaustive list of attributes. For example, organisms are described as more or less complex, more or less homeostatic, of different degrees of responsiveness to environmental variation in their physiology or development. In this way the changes in the system, which might require a very large number of dimensions to enumerate, are reduced to a scale of much lower dimensionality. At the same time the unordered, extensive descriptions of the system become ordered descriptions along a scale of complexity, homeostasis, environmental buffering, and so on.
A major problem of evolutionary theories is to decide on the scales that the evolved states of a system are to be ordered along. One form of evolutionary ecology is the theory of succession, which asserts that over relatively short periods of time, of the order of generations, the species composition of a community of organisms will undergo predictable changes. In specific regions this succession can be described simply by listing the plant species and noting their relative abundance at successive stages. In New England an abandoned farm field is first occupied by various herbaceous weeds, then by white pines; later the white pines give way to beeches, birches, maples, and hemlocks. This description is nothing but a list of changes. Attempts have been made to introduce order into the theory of succession by describing, among others, changes in (1) the total number of species; (2) species diversity, taking account both of the number of species and of their relative abundance; (3) biomass diversity including both the physical size of each species and its relative abundance; or (4) the ratio of total rate of production of living material to the total standing crop of material. None of these measures contains the actual list of species or their unique qualities, but rather establishes a single quantitative dimension along which community compositions can be ordered. There is, however, no a priori criterion for deciding which of these, if any, is a “natural” or even an empirically useful dimension. To choose among them it would be necessary to have a kinematic description of the evolution of the community that could be phrased in terms of the chosen dimension. That is, given some set of dimensions that describe the state of the system, E (t) at time t, it must be possible to give a law of transformation, T, that will carry E (t) into E' (t + 1): E' (t + 1) = T [E (t)].
But the search for a law of transformation cannot be carried out without some idea of the appropriate dimensions of description of the system, since there is no assurance that one can find a law by beginning with an arbitrary description. The development of a law of evolutionary transformation of a system and of the appropriate dimensions of description is a dialectical process that cannot be carried out by a priori assumptions about either law or description. In evolutionary ecology rather little progress has been made in this process precisely because the ordered states of description of communities have been chosen arbitrarily for their intuitive appeal rather than by any constructive interaction with the building of a kinematic theory. Evolutionary genetics has been more successful in building kinematic theories of evolutionary change, but only because it has given up the attempt to introduce an ordered description. Evolutionary or population genetics has an elaborate theory of the change in the frequency of genes in populations as a consequence of mutations, migrations, breeding structure of populations, and natural selection. But this theory is framed entirely in terms of an extensive list of the different genetic types in the population and how that list changes in time. Every attempt to find some ordered description of a population, as for example its average size, its reproductive rate, or the average fitness of individuals in it, whose transformations over time can be described by a kinematic law, has failed. Thus when the population geneticist Dobzhansky, described evolution as “a change in the genetic composition of populations” (1951, p. 16), he did so for lack of anything better. Described in this way, evolution is nothing but the endless reshuffling of the four basic molecular subunits of DNA.
The requirement of order marks the division between the purely mechanistic descriptions of evolutionary processes, as represented by Dobzhansky’s dictum, and those with some metaphysical element, leading in the extreme to the creative evolutionism of Bergson and Teilhard de Chardin. To assign the successive states of an evolutionary sequence to some order requires a preconception of order, a human conception that is historically contingent. In the case of the deck of cards, all sequences are equally probable, so any given completely mixed hand at poker has exactly the same probability as a royal flush; yet we are surprised (and rewarded) when a royal flush appears. Ideas of order are profoundly ideological, so the description of evolution as producing order is necessarily an ideological one. In this sense evolution is neither a fact nor a theory, but a mode of organizing knowledge about the world.
Direction
If an ordered description of the states in an evolutionary process has been created, it becomes possible to ascribe a temporal direction to evolution. Evolutionary processes are then described as the unidirectional increase or decrease of some characteristic. In one form of evolutionary cosmology the universe is said to be constantly expanding; in thermodynamics entropy increases. In evolutionary ecology it has been variously asserted that complexity, stability, or the ratio of biomass to productivity increases in time and that species diversity decreases or increases toward an intermediate value. In evolutionary genetics the reproductive rate of the population, its average size, and its average fitness have all been proposed as monotonically increasing with time, while for the evolution of life over geological time, it has been suggested that organisms are becoming more complex and more physiologically homeostatic. The history of human culture is described not simply in terms of the change from hunting and gathering to primitive agriculture, from feudal agriculture to capitalist industry. Instead, the modes of organization of production are placed on a graded scale as, for example, the degree of division of labor (Durkheim) or the degree of complexity (Spencer). Only historical geology has been largely free of a theory of monotonic evolution. The processes of orogeny and erosion by which mountains are raised and then slowly leveled by wind and water into a flat, featureless plain, only to be raised again in another oro-genic episode, form a repeated cycle with no general direction. Glacial and interglacial periods cyclically follow each other, causing the raising and lowering of sea level and a long cycle of temperature change. The recent theory of plate tectonics, according to which lava wells up to the earth’s surface along major cracks under the ocean, is also cyclic, since the spreading of the sea floor causes the opposite edges of the major lithospheric plates to slip downward (subduction) into the earth interior, where the material is again melted down. It is assumed that the total amount of the earth’s crust remains more or less constant in the process. Of course, in the very long term, the earth as a whole must cool, and all geotectonic processes must eventually come to an end, but the extremely long time scale of this prediction takes it out of the domain of geology proper, displacing it to the borderline with cosmology and thermodynamics, which have their own general theories of directionality.
The search for a direction in evolution is closely linked with the postulation of order. Indeed, the choice of the appropriate ordered description of evolutionary states is largely the consequence rather than the precursor of decisions about direction, although in some few cases the description of an evolutionary process as unidirectional along some set of ordered states may simply be a restatement of the underlying dynamic equations of the process. In classical physics the laws of the motion of bodies can be restated, by changing the parameters, as laws of the minimization of potential energy. Even more generally, movements of bodies and classical optics can both be subsumed under Fermat’s principle of least action, and nineteenth-century physics textbooks were sometimes cast in these terms.
Borrowing from this tradition in physics, evolutionists have looked for ways to parameterize the equations of population ecology or population genetics so that they will appear as maximization or minimization principles. Fisher’s fundamental theorem of natural selection (1930), showing that the average fitness of a population always increases, was such an attempt. Unfortunately the theorem turned out to be somewhat less “fundamental” than claimed since it applies only in specially restricted cases. In like manner ecologists have attempted to find in the equations of species interactions principles of minimization of unused resources or maximization of efficiency but, like Fisher’s fundamental theorem, such restatements apply only in special cases. When such principles have been stated, the mathematical result has been reified, and the consequent claims about the material world have often been confused or incorrect. The principle that the genetic changes in a population under natural selection result in an increase in the mean fitness of the population, even in the special circumstances where it is true, is only a statement about the relative fitnesses of individuals within the population and makes no prediction at all about the absolute survival and reproduction of the population. In fact, after undergoing natural selection a population is not likely to be more numerous nor to have a higher reproductive rate than before; it may even be smaller and have a lower reproductive rate. Yet the principle of the increase of relative fitness has been reified by evolutionists who suppose that species become, in some sense, absolutely more fit by natural selection (see Chapter 2).
More often the kinematic equations of evolutionary processes cannot be recast in terms of a directional change in some intuitively appealing ordered variable or, even more often, no kinematic equations exist for the process. Among all evolutionary processes only genetic evolution within populations and statistical thermodynamics have well-founded mathematical structures. Other domains, such as evolutionary ecology, are highly mathematized, but the dynamics on which their mathematical structures are based are entirely hypothetical, and thus their theories are elaborate fictions, which nevertheless may contain many truths. In the absence of an exact theory of evolution, directions are ascribed to evolutionary processes a priori, based on preexisting ideological commitments.
The scale most often appealed to is complexity. It is supposed that during organic and social evolution organisms and societies have become more complex. Spencer (1862) in his First Principles declared that the evolution of the cosmos, of organic life, and of human society all progress from the homogeneous to the heterogeneous, from the simple to the complex. Modern evolutionists largely agree. Vertebrates, and mammals in particular, are regarded as more complex than bacteria, and since the vertebrates evolved later than single-celled organisms, complexity must have increased. The brain is thought to be the most complex organ, so the human species, with its exceedingly complex brain, must represent the most advanced stage in evolution. Closely tied to the idea of increasing complexity is the theory that modern organisms contain more information about the environment than primitive ones, information stored during the evolutionary process in the complex structures of advanced species. Finally, the supposed increases of information and complexity are regarded as exceptions to the second law of thermodynamics, which requires a general increase in entropy and homogeneity, with a decrease in complexity by randomization. Evolutionists speak of the accumulation of “negentropy,” of complexity and information, as the unique property of living systems, marking them off from the inorganic.
The supposed increase in complexity and information during evolution does not stand on any objective ground and is based in part on several confusions. First, how are we to measure the complexity of an organism? In what sense is a mammal more complex than a bacterium? Mammals have many types of cells, tissues, and organ systems and in this respect are more complex, but bacteria can carry out many biosynthetic reactions, such as the synthesis of certain amino acids, that have been lost during the evolution of the vertebrates, so in that sense bacteria are more complex. There is no indication that vertebrates in general enter into more direct interactions with other organisms than do bacteria, which have their own parasites, predators, competitors, and symbionts. And even if we are to accept sheer structural variation as an indication of complexity, we do not know how to order it, not to speak of assigning a metric to it. Is a mammal more complex structurally than a fish? Yet 370 million years passed between the origin of the fishes at the end of the Cambrian and the first mammals at the beginning of the Cretaceous. If one starts with the assertion that structural complexity has increased, it is possible to rationalize the assertion a posteriori by enumerating those features, for example, a very large hindbrain, that appear later in evolution and declaring them to be more complex. The evident circularity of this procedure has not prevented its widespread practice.
A second difficulty with complexity as a direction in evolution arises from the confusion of modern “lower organisms” with ancestral organisms. Modern bacteria are not the ancestors of modern vertebrates, they are the product of more that a thousand million years of cellular evolution. While structurally more complex forms may have appeared later in the evolutionary sequence and evolved from less complex ones, they have not replaced the less complex but coexist with them. Evolution cannot be the change from less to more complex in general, because that description says nothing about the millions of years of evolution within grades of organization. The same confusion exists in anthropology. Modern “primitive” people are not the ancestors of “advanced” civilizations, and we do not know what the social structure was in the prehistoric ancestral human groups. The Bushmen of the Kalahari have as long a history as any other human group, so the judgment that their society is less evolved than ours requires making an a priori decision about the succession of stages that are to be taken as a description of social evolution and postulating that some groups have become arrested in their social evolution. The scale of comparison then ceases to be a temporal sequence and becomes instead a contemporaneous scale ordered by time of first historical appearance. The difference between contemporaneous grades based on time of first appearance and strictly historical sequences marks off organic evolutionary theory from a social theory like historical materialism. Nothing in the theory of organic evolution demands the replacement of earlier grades by later grades of organization, and some strong theoretical reasons from ecology suggest that coexistence is to be expected. In contrast, Marxist historical theory predicts the eventual replacement of one mode of production by another universally, although for long periods different, contradictory modes may coexist.
A third difficulty is the equation of complexity with information. It is not at all clear how the information in a structure is to be measured. The only concrete suggestion for organisms is to regard the genes as a code made up of three-letter words with a four-letter alphabet, then calculating the information in the total genetic “message” for each organism by the Shannon information measure. However, by this measure many invertebrates turn out to have more information than many vertebrates, and some amphibia are more complex than Homo sapiens. The problem is that complexity and information have only a metaphorical, not an exact, equivalence. While it is appealing to speak of “information” about the environment being “encoded” in the structural and physiological complexity of organisms, such statements remain in the realm of poetry.
The confusion is further compounded by the relation of metaphorical notions of complexity and information to the second law of thermodynamics. “Entropy,” which is the Greek equivalent of the Latin “evolution,” was introduced in the nineteenth century as a property of the universe that is always increasing. In the original macroscopic form of thermodynamics, it meant simply that different regions of the universe become, in time, more and more alike in their mean energy levels, so less and less useful work can be derived from their interaction. The kinetic theory of gases and, later, statistical mechanics reinterpreted this principle to mean that in any defined region of space the kinetic energy of molecules would eventually have the same distribution as in any other region of space, because connections between the regions would lead to a randomization of the molecules and a redistribution of energies through collisions. Evolutionists have incorrectly interpreted this theory to mean that all molecules would have the same kinetic energy rather than that collections of molecules would have the same distribution of energies. Moreover, they have confused kinetic energy with gravitational and electromagnetic energy and have supposed that a general second law guarantees that all the molecules in the universe will eventually become equally spaced out into a formless and orderless final state. Given the belief that the physical universe is moving toward a static death rather than a thermodynamic equilibrium in which molecular motion continues, it is no surprise that evolutionists believe organic evolution to be the negation of physical evolution. In actual fact, whatever its other properties, the evolution of organisms must accord with the entropic changes in the physical universe. At present living organisms exploit, for their maintenance and reproduction, the differences in kinetic energy between regions of space; and at the same time they contribute to the increase in entropy. Life cannot exist without free energy and is constrained in its evolution by thermodynamic necessity.
Evolutionary thermodynamics, with its directionality embodied in the second law, is superficially similar to another directional cosmology, the theory of the expanding universe. According to this cosmogony, the universe came into being more than ten thousand million years ago in a restricted region of space, all matter having been created in an initial explosive burst. Matter continues to expand in all directions around the point of origin, so as time goes on the universe will become more and more thinly spread out. This spreading is only global, however, and does not mean that individual clumps of matter like planets will necessarily break up. Thus the expanding-universe cosmology, while directional, also has specific historical content, in that the accidental accumulations of matter resulting from the original unique event will remain permanently in existence, held by their gravitational and electromagnetic forces.
Even in thermodynamics and cosmology, however, the assertions of uniform direction have been challenged. Recently, Bondi, Hoyle, and Gold have proposed nondirectional theories of the cosmos. In one such theory matter is constantly being created anew, so that despite the expansion of the universe, the average density of matter remains the same. An alternative is a cyclic expansion-contraction theory, producing an oscillating universe with a very long cycle time. In thermodynamics it has been postulated that entropy may be increasing only locally, that in other regions of space it may be decreasing, and that the universe as a whole is in a steady state.
The suggestion that organic evolution leads to an increase in complexity is closely tied to the concept of homeostasis, introduced by Cannon in 1932 as a general principle of physiology. Organisms have a variety of physiological, structural, and behavioral characteristics that result in the maintenance of certain physiological states at a constant level despite environmental fluctuation. Mammals maintain a constant body temperature over a very wide range of ambient temperatures by varying their metabolic rate, dilation of blood vessels, erection of body hair, sweating, panting, and so on. In general, homeostasis is the maintenance at a constant level of those characteristics whose constancy is essential to survival, by varying other characteristics in response to signals from the environment. Evolutionary ecologists have extended the concept of homeostasis to entire assemblages of species that are related to each other by predation and competition. If grass becomes sparse because of fluctuations in rainfall, herbivores will reproduce at a somewhat lower rate, but their predators will also be reduced by the lower abundance of prey, and the net effect will be to stabilize the community at somewhat lower numbers temporarily without any species becoming extinct. Homeostasis is thought to increase in evolution because it results in stability, and dynamic stability, the return of a system to its previous state after a perturbation, is seen as the outcome of all dynamic processes.
It is through stability that complexity and homeostasis become connected in evolutionary theory. Virtually every modern theorist of evolution, especially evolutionary ecologists, has claimed that complexity results in stability. Complexity, in turn, is thought of as a consequence of strong interactions among many diverse elements with different functions. For example, it has long been supposed that a community of species with many different predators, competitors, decomposers, and primary food sources, all strongly interconnected in their population dynamics, is the most resistant to the effect of perturbations in the environment. This means that diverse assemblages of organisms are more stable, so diversity is also seen as a direction of evolution. The entire metatheoretical structure of present-day evolutionary theory consists in the interconnection of these concepts. Diversity of form and function together with strong interdependence of the diverse elements are the components of complexity, which in turn leads to stability through greater homeostasis. Evolution leads to greater and greater diversity, complexity, homeostasis, and stability of the living world, in a physical environment that is increasingly uniform, simplified, and chaotic.
The extraordinary feature of this conceptual structure is that it has no apparent basis either in fact or in theory. We have already shown the problems of measuring complexity and demonstrating its increase during evolution. From the beginning of life on earth, diversity certainly has increased, in that the variety of organisms, both in the number of species and in the kind of habitats they occupy, is greater now than it was in the Cambrian. But it has been about 350 million years since vertebrates invaded the land and 150 million years since they first exploited aerial environments, while insects occupied both land and air at least 300 million years ago. Different groups have reached their peaks of diversity, as measured by the number of genera or families, at different times, and taking the fossil record as a whole, no apparent increase in overall taxonomic or ecological diversity has occurred for the last 150 million years. Long-term trends toward diversity do appear for particular groups, for example the slow but steady increase in the number of families of bivalve mollusks over the last 500 million years. But such trends are in part an artifact of taxonomic practice, in part the result of the greater likelihood of finding more recent fossils, and in part the result of the breakup of the single large continent, Pangaea, beginning about 250 million years ago, which created a much greater diversity of marine habitats as a temporary historical fact. If the present continents drift together again, the diversity of marine mollusks will inevitably decrease. On the time scale of ecological rather than evolutionary changes, one finds both increases and decreases in diversity in the succession of species composition in temporarily disturbed terrestrial and aquatic environments. Certainly, no empirical generalization is possible. At the theoretical level the situation is even more extraordinary. Despite the repeated claim that greater complexity and diversity lead to greater stability, no rigorous argument has ever been offered in support of this theorem and, on the contrary, recent mathematical and numerical studies in both the theory of community ecology and in population genetics have shown exactly the opposite to be true. If complexity of a community is defined as the number of species interactions multiplied by the strength of the interactions, it has been shown that as this complexity increases, by adding more species or by increasing the strength of the interaction, the probability that the community will be stable to perturbation decreases rather than increases (May, 1973).
The emphasis on diversity, complexity, and stability as the trends in evolution can only be understood as ideological in origin. While change and motion were the intellectual motifs of the bourgeois revolution, as a legitimation of the overturning of old class relations, the consolidation of that revolution in the latter part of the nineteenth and in the twentieth century has required a different view, consonant with a newly stabilized society. Change had to be tamed in science as it was in society. The result has been an emphasis in modern evolutionary theories on dynamic stability.
Although individual elements in the system are changing place, the system as a whole remains in a steady state; in the same way individuals may rise and fall in the social scale, but the hierarchy of social relations is thought to be unchanging. For social theorists the bourgeois revolution was the last step in a social evolution away from artificial and unstable hierarchies to a natural social structure based upon the free movement of individuals according to their innate abilities. The society that has been produced is one of great complexity, with an immense division of labor and very strong interactions among the component parts. Moreover, the stability of the modern social order is thought to be provided precisely by the complex interactions among the individual units, each dependent upon the others. The description of the evolution of biological systems is a mirror of the supposed evolution of modern bourgeois society. An ironic result of this view of evolution has been that the environmentalist movement of the present day has used the preoccupation with stability and complexity of natural communities of species to oppose the expansion of the very capitalist system of production that gave rise to the ideology originally.
In the twentieth century there has been a general change of emphasis from directionality to steady-state theories of evolution. In cosmology the perpetual-creation theories and the expansion-contraction theory postulate that the universe is in a long-term steady state or a cyclic oscillation. Thermodynamic theories allow that entropy may be increasing only locally in space-time. Theories of organic evolution are now entirely preoccupied with stability and dynamic equilibrium. The literature of theoretical evolutionary genetics and evolutionary ecology is almost totally taken up with finding the conditions of stable equilibrium of genes and species or with trying to distinguish different special theories of phenomena on the assumption that they are in stable equilibrium. The chief controversy in evolutionary genetics for the last thirty years has been whether the observed genetic variation among individuals is maintained by natural selection or is the consequence of repeated mutations of unselected genetic variants. Proponents of both schools depend upon elaborate mathematical analysis and statistical treatment of data on the assumption that populations in nature are in an equilibrium state, with no trace of their past histories. Like modern bourgeois social thought, modern evolutionary thought denies history by assuming equilibrium.
The emphasis on equilibrium must nevertheless accommodate the obvious fact that evolution continues to occur. There is no trace in the fossil record that the formation and extinction of species have ceased or even slowed down, and rates of morphological change within evolutionary lines remain high, even in the most recent fossil horizons. If evolution and adaptation continue to occur, how can the world be in a steady state? The answer given is that the environment is constantly changing, always decaying with respect to the current adaptation of species. In this view the continued evolution of organisms is simply keeping up with the moving, worsening environment, but nothing is happening globally. The environment worsens because resources are used up, because competitors, predators, and prey evolve, and because any change makes previous adaptations obsolete. No species can ever be perfectly adapted because each is tracking a moving target, but all extant species are close to their optima. Species become extinct if they evolve too slowly to track the moving environment or disperse too slowly to keep up geographically with their preferred environment. In this way modern evolutionary theory solves the apparent contradiction between the observation of continued evolution and the ideological demand that the assemblage of organisms be stable and optimal.
Progress
The view of nineteenth-century evolutionists was quite different. For them evolution meant progress, movement from worse to better, from inferior to superior. The idea of progress requires not only a theory of direction but also a moral judgment. Even if it were granted that organic evolution resulted in an increase in complexity, that trend would not be progressive unless some general theory of value made it so. The moralism in ideas of evolutionary progress is seldom made explicit but is usually hidden in the assumption that the human species represents the highest and best form of nature. Most modern evolutionists have tried to expunge anthropocentric moralism from their theories, but a few, such as Teilhard de Chardin, have reverted to nineteenth-century progressivism. For Teilhard de Chardin (1962), “Man is the only absolute parameter of evolution,” by which he means not merely organic but cosmic evolution. This is an echo of Whitehead’s (1938) division of occurrences in nature into six types, of
which “human existence, body and mind” is the highest, other animals are next, plants the next, and so on down to atomic particles. Man leads all the rest. The shibboleths of progressivism are the superiority of man in the cosmos, of industrial man in the world economy, and of liberal democratic man in world society. We have, then, a kind of Whig biology, which sees all of evolution as leading to entrepreneurial man.
The most influential spokesman of evolutionary progress in the nineteenth century, Herbert Spencer, equated progress with change itself. Spencer claimed that: “From the earliest traceable cosmical changes down to the latest results of civilization, we shall find that the transformation of the homogeneous into the heterogeneous is that in which progress essentially consists” ([1857] 1915, p. 10). He believed that this transformation had occurred in the arts, in forms of political organization, in language, in economic relations, and in the history of organic life. But Spencer did not offer any other justification for the progressive quality of change. For him change of any sort and in any direction was by its nature progressive, “a beneficent necessity.” The contrast between Spencer’s belief in the intrinsic progressivism of change and the present belief in stability and dynamic equilibrium, with species fighting a rearguard action against a threatening environment, is the contrast between the optimistic, revolutionizing bourgeoisie committed to destroying the old restrictive social relations in the nineteenth century and an entrenched but embattled capitalism asserting its stability and permanence in the face of a deteriorating world situation in the twentieth.
There is another sense in which evolutionary sequences are regarded as progressive and in which the moralistic element comes directly from economic ideology. Darwin laid special emphasis on the “perfection” of organs like the eye, with its complex arrangements for focusing, varying the amount of admitted light, and compensating for aberration, as a severe test of his theory of evolution by natural selection. Evolutionary theory was meant to explain not only the manifest diversity of organisms, but also the obvious fact that the organisms showed a marvelous fit to nature. The concept of adaptation is that the external world sets certain “problems” for organisms and that evolution consists in “solving” these problems, just as an engineer designs a machine to solve a problem. So the eye is a solution to the problem of seeing; wings of flying; lungs of breathing. Putting aside the great difficulties of deciding what problem is posed by nature, or what problem a particular organ is a solution to (see Chapter 2), there is the question of deciding how good the solution is for a given problem. This requires a criterion of optimality, so one can judge how close to optimum the evolutionary process has brought the organism. Present evolutionary theory assumes that such optima can be specified for particular situations and that evolution can be described as moving organisms toward the optimal solutions. Because the problems are always changing slightly, no species is ever exactly at its optimum, but extant species have achieved near-optimal solutions and would improve their fit if the environment remained constant for a sufficient period. In fact, some forms of optimization theory, including the theory of games, have been taken over from economics and political science as techniques for prediction and explanation in organic evolution, replacing purely kinematic theories of population genetics and population ecology. In kinematic theories a few basic assumptions are made about the mechanics of inheritance or the elementary rules of population growth, a predictive mathematical or numerical machinery is constructed from these assumptions, and the trajectory of the process is predicted. Optimization theories have no kinematics. It is assumed that evolution carries a system to its optimum, the optimum is described, and the state of the system is compared to it.
Putting an optimization program into practice requires a general theory of optimality, which evolutionists have taken directly from the economics of capitalism. It is assumed that organisms are struggling for resources that are in short supply, a postulate introduced by Darwin after he read Malthus’s Essay on the Principle of Population. The organism must invest time and energy to acquire these resources, and it reinvests the return from this investment partly in acquiring fresh supplies of resource and partly in reproducing. That organism is most successful which acquires the greatest net surplus for investment in successful reproduction. There are then two criteria of optimality. One is the expenditure of the least amount of energy for each unit of resource acquired and the other is the allocation of the largest proportion of acquired surplus to reproduction, subject to the requirement that sufficient surplus is available for new acquisition. In practice the criteria for these problems of optimum resource allocation are minimum time or maximum yield. An example is the problem of time allocation in birds that gather food and bring it back to the nest to be consumed (central-place foragers). In nature food particles vary somewhat in size. If the bird searches only for the largest particles, this may be so time consuming that the energy of searching and return is not sufficiently repaid. On the other hand, if the bird takes the first particle encountered, it may be so small that again the net return is too small. In theory the bird will choose particle sizes that will maximize its net return per unit time. However, spending long periods away from the nest leaves the young vulnerable to predators, so some proportion of time must be invested in guarding the nest. The optimizing theory of evolution assumes that time allocation will be close to optimal for maximizing total investment in reproduction, or growth of the firm. In such theories the criterion of optimality is efficiency, whether of time or invested energy, yet the moralistic and ideological overtones of “efficiency,” “waste,” “maximum return on investment,” and “best use of time” seem never to have come to the consciousness of evolutionists, who adhere to these social norms unquestioningly.
Perfectability
Darwin’s contemporaries believed that evolutionary progress led to “that perfection of structure and coadaptation which justly excites our admiration” (Darwin 1859, p. 3). At the conclusion of Origin of Species Darwin wrote that “as natural selection works solely by and for the good of each being, all corporeal and material endowments will tend to progress toward perfection” (p. 489), yet earlier he was cautious: “Natural selection will not necessarily produce absolute perfection; nor as far as we can judge by our limited faculties can absolute perfection be found predicated” (p. 206). Darwin knew that universal perfection of adaptation required that the necessary variation must arise and that the organism’s relationship to the environment must remain constant over a long period. Since he knew that neither of these conditions is unfailing, he realized that perfection is not inevitable, although characters “tend to progress toward perfection.” Thus Darwin stopped short of the Panglossian view that evolution has resulted in the “best of all possible worlds,” a view that characterizes much of the adaptational thinking of twentieth-century evolutionism.
Because of the theory that the environment changes, perfectability does not imply that evolution will cease when perfection is reached. The perfectly homeostatic organism that could survive better than any specialized form in every environment is regarded as a biological impossibility, although it is sometimes postulated that the human species, with its faculty for culture, may be such an organism. Outside the domain of biology, evolutionary theories do include the postulate of a final state to which the system is converging. In nonsteady-state thermodynamics, entropy is increasing everywhere, and eventually the universe will approach a steady state of maximum entropy in which no work can be performed by thermal interaction. More general directional cosmological theory makes the same prediction for an expanding universe, including radioactive and thermonuclear forms of energy. Evolutionary theories of social systems, specifically Marxism and some of its variants, are explicitly progressivist and perfectionist. A stage of primitive capital accumulation through piracy, the exploitation of slaves, serfs, and the cheap resources of outlying regions, is succeeded by a bourgeois revolution and the breaking of feudal and slave relations through the introduction of liberal democracy. The resulting unleashing of productive forces in turn leads to proletarian revolution, proletarian democracy, and an eventual elimination of social classes. Some differentiation of individuals will persist in social activity and personal life pattern: “From each according to his abilities, to each according to his needs,” but the entropy of the social system is maximized with respect to the categories of economics. The parallel with thermodynamic equilibrium is striking.
Darwin and evolution
The Background of Darwinism
To understand the development of the modern theory of organic evolution, it must first be realized that Darwin was the culmination and not the origin of nineteenth-century evolutionism. In 1859, when Origin of Species was published, the evolutionary world view already permeated natural and social science. Evolutionary cosmology was founded in Kant’s Metaphysical Foundations of Natural Science of 1786 and in Laplace’s nebular hypothesis of 1796. Hutton’s principle of uniformitarianism appeared in 1785 and became the dominant view in geology as a result of its centrality in Lyell’s Principles of Geology of 1830. Evolutionary thermodynamics was begun by L. N. Sadi Carnot in 1824 and came to full development in 1851 in the work of William Thomson. In social science, Spencer’s influence was enormous. In 1857, in Progress: Its Law and Cause, he could claim that “it is now universally admitted by philologists, that languages, instead of being artificially or supernaturally formed, have been developed. And the histories of religion, of philosophy, of science, of the fine arts, of the industrial arts, show that these have passed through stages.” (p. 9). English literature of the first half of the nineteenth century was thoroughly imbued with evolutionist ideology. Around 1840 Tennyson wrote in “In Memoriam” that nature did not preserve “the type” but that “From scarped cliff and quarried stone/ She cries, ‘a thousand types are gone:/I care for nothing, all shall go.’ ” (part 56, stanza 1). His epic poem Idylls of the King established that “the old order changeth, yielding place to new.” Dickens described the destruction of the old order in Dombey and Son (1846) and Bleak House (1852) and made it the central theme of Hard Times (1854).
Biology was the last domain of intellectual life to incorporate the evolutionary world view, in part because it directly threatened ideas of the uniqueness and superiority of the human species. Nevertheless, the idea of organic evolution was widespread, if not dominant, before 1859. Charles Darwin’s grandfather, Erasmus Darwin, published Zoonomia in 1794 and The Temple of Nature in 1803, which expressed romanticized but remarkable prescient views of the origin and evolution of life, including man. Between 1794 and 1830 in France, Lamarck and Geoffroy Saint-Hilaire developed theories of organic evolution that contradicted the powerful Baron Cuvier’s attempt to explain the fossil record by repeated floods. Spencer, in 1857, argued for the evolution of life on the basis of the generality of the principle of evolution in every other domain. Darwin himself, in the third edition of Origin of Species in 1861, provided a historical sketch of the writings on organic evolution prior to his own.
Not only the intellectual realm, but the family and political milieu as well, reinforced the ideology of change and movement for Darwin. His maternal grandfather, Josiah Wedgwood, began as a poor apprentice and became a great industrial magnate, the very epitome of the new class of self-made industrialists. Darwin’s paternal grandfather, Erasmus, a selfmade man, belonged to the social circle of new midland industrialists that included Wedgwood, James Watt, James Keir, and Matthew Boulton. Charles’s father took forty pounds of Erasmus’s money and made himself well to do by his own activity, at a time when the high Tory prime minister was Robert Peel, grandson of a peasant turned peddler. Darwin set out on the voyage of the Beagle at the height of the agitation for the Reform Bill of 1832, and he had developed most of his ideas for Origin of Species by the time of the revolutions of 1848.
Darwinism and Materialism
The pervasiveness of evolutionism, resulting from the political economic revolution, led to a serious contradiction with an older intellectual tradition, inherited from Plato and Aristotle, that was consonant with the older static world order. This was the concept of the ideal type. According to this view, real objects in the world were imperfect manifestations of underlying ideal patterns. The ideals had no material form but could be glimpsed “through a glass, darkly” by studying real objects. The purpose of scientific study was to understand the ideal types, and the problem of science was to infer these types despite the imperfection of their manifestations in the world. A corollary of this typological approach is that the variation among objects of a given type is ontologically different from the variation among types. The differences among objects within a type are the result of “disturbances” which, although they may have some subsidiary intrinsic interest, are essentially a distraction, while the study of the types themselves will reveal the essential underlying structure of the universe. Newton’s ideal bodies moving in empty space were examples of types that abstracted ideal motion from real motion, putting aside friction, inelasticity, and the occupation of finite space by mass in order to construct the “basic” laws of motion. Each species of living organism was regarded as a “type,” and the actual individuals in nature were imperfect manifestations of the true species ideal. Even at present the type is still used in taxonomy; it is a single individual that is deposited in a collection and designated as the standard of comparison against which all other individuals thought to belong to the species are matched. Actual specimens vary from the type, and sometimes the type specimen turns out to be quite untypical of the species as a whole. Modern taxonomic practice has moved away from this tradition in part by designating holotypes, populations of specimens whose statistical properties are taken as representative of the species as a group.
The theory of ideal types established the problematic for pre-Darwinian evolutionary theory: how do organisms pass from one type to another or, alternatively, how can new types come into existence? The fact that all organisms were the offspring of other organisms made the problem even more difficult, since in the process of continuous reproduction some instant must mark the passage of living material from one type to another, or at some instant a new type must come into existence and be represented by a material form that at the previous moment had belonged to a different type. Two general solutions to this dilemma were offered, neither of which could be satisfactory from either a physical or a metaphysical standpoint. Lamarck’s theory was that organisms changed type slowly by the accretion of small differences during the individual’s lifetime. Thus the giraffe stretched its neck to feed on higher leaves, the offspring of this giraffe would have a slightly longer neck, which would in turn stretch to reach still higher leaves, and so on over time. The transformation occurred because the animal sensed the need for more food, and this need instituted a change in form that was an adaptive response. The resultant change had to be incorporated into the heredity of the organism. Since plants were not regarded as having such feelings of need, Lamarck did not apply the theory to them, which very much weakened its appeal even to his contemporaries.
Geoffroy Saint-Hilaire, in contrast, proposed that types changed suddenly at the time of reproduction by major jumps in structure, rather than by the re-creation of small changes. The motive force for these abrupt changes was not made clear, nor did the theory help to understand the obviously adaptive nature of the differences between organisms. One of the major arguments for divine creation of species was that organisms seemed designed to fit their environments. An acceptable theory of evolution would need to account for this fit as well as offer a convincing mechanism for the origin of new varieties. When Origin of Species appeared, the contradiction between change, as demanded by the evolutionist ideology and the Platonic-Aristotelian ideal of fixed types, had not been satisfactorily resolved.
Darwin’s intellectual revolution lay not in his theory that organisms evolved, since that was already widely believed, but in his rejection of Platonic-Aristotelian idealism and his total reorientation of the problematic of evolution. Instead of regarding variation among individuals as ontologically different from the differences among species, Darwin regarded differences within species and differences among species as ontologically related. He took differences among individuals as the primary object of study, concentrating on the real and material differences among the living organisms themselves. He replaced the ideal entities, species, with the material entities, individuals and populations, as the proper objects of study. Darwin’s revolutionary insight was that the differences among individuals within a species are converted into the differences among species in space and time. The problematic of evolutionary theory then became—and remains to the present day—to provide the mechanism for this transformation.
The Darwinian Theory
Once it is assumed that evolutionary change is the result of the conversion of variation among individuals into variation among species and of successive alterations of species over time, it is necessary to identify the force for that conversion and to describe the mechanism by which the force converts the variation. That is, we need a dynamics and a kinematics. Darwin supplied both.
The force postulated by Darwin was natural selection, which resulted from the struggle for survival. Darwin dated his concept of natural selection from his reading in 1838 of Malthus’s widely known Essay on the Principle of Population. Malthus’s argument was that human reproduction caused the population to grow geometrically, while the resources available grew only arithmetically, resulting in a struggle among people for resources in short supply. For both Darwin and Alfred Russel Wallace, who simultaneously developed theories of evolution by natural selection, Malthus’s human struggle was the model for all species. But the theory of natural selection arose independently from Darwin’s study of Malthus, as did the metaphorical term “natural selection.” Darwin began Origin of Species with the chapter “Variation under Domestication,” followed by the parallel “Variation under Nature.” “Variation under Domestication” served two functions. First, it illustrated, through examples of pigeons, cattle, and fruit trees, the immense variety of forms that is latent within a species, so that a parallel with the situation in nature could be drawn in the next chapter. Second, it explained how these diverse breeds were created by deliberate selection: “The key is man’s power of accumulative selection: nature gives successive variations; man adds them up in certain directions useful to him” (1859, p. 30). The concepts of variation and selection were thus intimately tied together through the consideration of domestication. The problem was then to provide an analogue to “man’s power of accumulative selection” to carry through the inference from domestication to nature. Here Darwin’s materialism again showed itself. Instead of postulating a mysterious force, a personified Nature, he derived the principle of natural selection from the struggle for survival that follows from overreproduction in a world of finite resources. He extended the analogy with human struggle beyond Malthus in the principle of sexual selection in which he appropriated the Victorian view of the relations between men and women. According to this theory males are in competition with each other to acquire females, both by being more attractive to them in courtship displays and by physically excluding competing suitors.
Darwin’s proposition of a direct material force by which “nature” can “select” among variations to produce more fit types, together with his concentration on individual variation as the proper object of study, created a mechanism for evolution that was in contrast to the mere explanations of Lamarck and Geoffroy Saint-Hilaire. The mechanism was contained in three propositions:
- Individuals within a species vary in physiology, morphology, and behavior: the principle of variation.
- Offspring resemble their parents on the average more than they resemble unrelated individuals: the principle of heredity.
- Different variants leave different numbers of offspring: the principle of natural selection.
From these three principles it follows mechanically that evolution will occur. Provided that offspring resemble their parents more than others, if a particular variant leaves more offspring than another variant, the composition of the population will change in the next generation. As time passes, the population will become more and more enriched with the variant that has a greater reproductive rate, and the species will change progressively. We thus have a kinematics of the evolutionary process.
The dynamics are provided by the struggle for existence. The reason some variants leave more offspring is that they are better able to appropriate resources in short supply and reinvest those resources in the production of offspring. This superior efficiency is a manifestation of their greater degree of engineering perfection for solving the problem set by the environment. The mechanism then accounts not only for change, but for adaptation as well. In contrast, neither Lamarck nor Geoffroy Saint-Hilaire provided more than ad hoc explanations. Although Lamarck’s inheritance of acquired characters was a possible mechanism for evolution, it had no empirical basis. Moreover, to provide for adaptive evolution, Lamarck required a metaphysical “inner urge” to fulfill needs. Geoffroy Saint-Hilaire’s theory of saltation could at least be substantiated by the occasional observation of a grossly different, although usually monstrous, variation in nature; however, these variant individuals played no role in the theory because there was no mechanism for passing from individual variations to transformations of species. If somehow the variant form were to reproduce its own kind, a new species would be formed, but no alteration in existing species would follow from such a postulate.
Darwin’s theory, remarkably, was devoid of any inferred but unobservable entities such as forces, fields, or atoms. There were no abstracted and idealized bodies moving in ideal paths from which real bodies departed more or less. The Newtonian revolution of the seventeenth century, in which idealization played a central and essential role, was totally removed in spirit and method from the Darwinian revolution of the nineteenth century, which was accomplished precisely by clearing away metaphysical concepts and concentrating on the actual variety among natural objects.
The theory of selection among variations is in itself incomplete as an explanation of evolution. First, it does not deal with the origin of the variation, which turned out to be an exceedingly embarrassing problem for Darwin. If selection causes the differential reproduction of variants, eventually the species population should uniformly be the most fit type among those available at the start. But then there would be no more variation for further evolution. Darwinian evolution by selection among variants is a self-negating process, which consumes the fuel, variation, on which it feeds and so destroys the condition for its further development. To suppose that evolution had continued for millions of years and would continue in the future required either the patently absurd postulate that all variations ever selected in the history of life were present from the beginning, or else that a mechanism existed to generate fresh variation. Darwin dealt with this problem only in generalities, claiming that altered environmental conditions evoked variations specific to the kind of organism and implying that such induced variations were heritable.
Second, even if there had been a mechanism for the origin of variation, Darwin established no mechanism for its inheritance. He vacillated about the nature of inheritance. In Variation of Animals and Plants under Domestication (1868), he put forward the “Provisional Theory of Pangenesis,” which postulated large numbers of unobserved “gemmules” that budded off from various organs and somehow assembled in the reproductive organs. The theory of natural selection did not require a detailed mechanism for inheritance except, once again, for the problem of variation. Darwin believed in blending inheritance, according to which the characters of the offspring were intermediate between those of its parents. But such a means of inheritance would very rapidly reduce variation in the species as a direct consequence of sexual reproduction, just as mixing different colored pigments soon leads to a single uniform hue. No satisfactory solution to this contradiction was produced until the rediscovery in 1900 of Gregor Mendel’s experiments.
Third, the theory of selection among variations can explain the slow transformation of a single species in time, but it cannot, in itself, explain the splitting of a species into diverse lines. To explain diversification, it was necessary to add statements about the geographical distribution of species. If some members of a species colonize a new and distinct habitat such as an island, where environmental conditions differ from those the species is used to, natural selection will produce a new variety there and, as a result of its new adaptations, the island variety may no longer be able to interbreed with the main population. Darwin considered this process of speciation by geographical isolation, presumed at present to be the chief process of diversification, (Mayr 1963) as particularly important. He also postulated that speciation would occur in organisms spread over large areas with many diverse ecological situations, even if there were no sharp geographical boundaries between local populations. Modern evolutionary theory puts rather less emphasis on the process of speciation in such quasi-continuously distributed species, but speciation has undoubtedly occurred under such circumstances.
Genetics and evolution
Races and Species
The Darwinian theory of evolution was that the variation among individuals within a population was converted to the differences among populations in time and space. The genetic theory of evolution specifies three modes of this conversion. First, selection within a population decreases the variation by increasing the frequency of one of the variants in time, changing the population composition and the distribution of characters. Second, in different parts of a species’ geographical range, the selection of different genotypes causes divergence among populations. In the process the variation within regions decreases, while the differentiation among regions increases. Third, genetic drift causes a loss of genetic variation within a population, but because it is on the average nondirectional, different populations become enriched for different genotypes as differentiation in space occurs. In addition, genetic drift may promote alternative outcomes of a single selective process, which once again increases the divergence among populations as the variation within populations decreases. In all cases the different genotypes that come to characterize the temporally or spatially differentiated populations were at one time components of the genetic variation within populations. It is this sorting out of an originally heterogeneous population into separate, more homogeneous assemblages that seems antientropic.
The differentiation of populations in space depends upon a sufficient restriction of migration that the centrifugal forces of genetic drift and differential selection are not swamped by the randomization process. If the populations are totally isolated from each other, as on different islands, there is no limit, except the availability of genetic variation, to the divergence that may occur. In modern evolutionary theory, two populations that differ in the frequency of any gene are called geographical races. Since every population that is partially isolated is likely to differ to some small extent, this view makes geographical race and local population essentially synonymous. If differentiation is somewhat greater, alternative gene alleles may be essentially pure in different populations, so individuals are recognizably characteristic of their population. This stage of divergence corresponds to the taxonomic category of subspecies, but in reality it is only an extreme form of geographic race. Indeed the division of population differentiation into distinct states is a vestige of the older typological view and bears no clear relation to the continuous process of evolutionary differentiation. Divergence in isolation may then become sufficiently great that the passage of genes between populations becomes biologically impossible because hybrid offspring fail to survive or because there are morphological, physiological, or behavioral barriers to mating. At that stage the populations are species, whose future evolution is genetically independent, except insofar as they may interact as competitors in a community of species. If the two newly formed species come into contact early in the speciation process, selection will generally reinforce the barriers to mating, since individuals who waste their gametes in forming inviable or sterile hybrids will leave fewer offspring.
This rather general description of the accumulation of genetic differences among isolated populations until they are so differentiated as to be species is all that present genetic evolutionary theory has to say about the origin of species. We have no knowledge of the actual nature of the genetic differences among species at any stage of their divergence. Do hybrid inviability and sterility involve only a few genes, or is there a wholesale differentiation across the genome? Is most speciation the direct consequence of selection for different ecological relations, or is it an accidental stage in a general process of genetic divergence? What is the relative speed of the initial divergence as compared with the speed during the period of reinforcement of reproductive isolation? We do not know.
There is yet a deeper difficulty. The genetic description of species formation is a purely mechanical specification (and a rather vague one) of how a single interbreeding population becomes broken into two reproductively isolated groups. But no account is taken of the fact that speciation involves the formation of a new biological entity that must interact with other species and become part of an ecological community. The genetic description of speciation asserts the occurrence of diversifying selection without describing its content. It is a kinematics without a dynamics. It does not cope with the problem of transforming the quantity of genetic change into the quality of being a new biological species and not just another gene pool. This failure of evolutionary genetic theory arises because the theory is totally formal in nature and because it reduces all qualitative forms of the interactions of organisms with each other and with the physical environment to the single quantitative variable, fitness.
Novelty and Adaptation
In the decades following the acceptance of Darwin’s theory, doubts lingered about the efficacy of natural selection for the production of anything new. It was generally acknowledged that selection could reduce the frequency of gross abnormalities in populations, eliminate inviable types, and therefore increase the frequency of better adapted types. But, it was argued, would it really reduce the frequencies of parts having only a slight disadvantage, or only those with gross deficiencies? Would selection only preserve what was already present? Could it introduce anything new?
A partial answer was provided in the 1920s and 1930s by the development of mathematical population genetics. J. B. S. Haldane, R. A. Fisher, and Sewall Wright formalized the Darwinian principles and the rules of Mendelian genetics into a quantitative theory which showed that even with very weak selection and a small mutation rate, populations could change drastically on a time scale much shorter than evolutionary time. They were able to conclude that if something new arises by mutation and if it confers even a small advantage, it will replace the previous types in a population.
The power of the mathematical theory, and its experimental vindication in studies of laboratory populations and in plant and animal breeding led to its general acceptance as a model not only of the short-term adaptative processes that could be monitored, but also of macro-evolution. In the “new synthesis” of evolutionary theory of the 1930s and 1940s, macroevolution was considered to be microevolution continued for a longer time. The problem of the origin of novelty was then answered as follows:
- New traits arise from random mutation.
- Rare genes become frequent through selection, and different rare genes, after being selected, come together to produce new combinations, which produce characteristics not previously seen.
- A higher-order randomness due to accidental fixation of even nonadvantageous genotypes in small populations produces interpopulational variation that may be selected and that also determines the background against which selection continues. (This was Wright’s special contribution.)
However, the approach of quantitative genetics remained isolated from both developmental biology and ecology. Phenotypes and environments entered only formally as coefficients; nothing was said about what kinds of novelties might arise, or under what circumstances they might be advantageous. We were left mostly with a theory for the quantitative improvement of preexisting adaptations.
Some authors, including Richard Goldschmidt (1940), concluded that the quantitative changes of ordinary responses to selection could not account for novelty. He therefore argued that macroevolutionary events are different in kind from the familiar adaptive processes of microevolution. Although they are random events, they are not of the kind that yields ordinary mutational variants. Rather, he saw the source of change in the radical reorganization of the genetic system, altering the whole pattern of development. These macromutations would usually be inviable, but in the rare instances where they conferred an advantage, they would initiate a whole new evolutionary direction. Goldschmidt was most successful in criticizing the inadequacy of the gradualist, continuous model of evolution. But his alternative theory did not really solve the problem. Even if his idea of macromutations was correct, the theory did not address what kinds of variations could possibly arise or even what kinds would be viable. The problem of the creativity of evolution remained: the origin of qualitative change from quantitative change.
The Marxist-Hegelian idea that qualitative changes could arise from quantitative change ran counter to the mechanistic materialism that predominated in the working ideology of scientists. In the mechanistic world view, changes in position, amount, velocity, and intensity were directly understandable, provided the intermediate stages could be shown, but discontinuous or qualitative change was mysterious. Darwin believed that “nature does not take jumps.” He therefore sought as the strongest evidence for his theory the existence of intermediate forms and admitted as damaging the absence of intermediates and the incompleteness of the fossil record. Subsequently biologists, compelled to accept the evidence of evolution by descent with modification, searched for ways of seeing evolutionary change as only epiphenomenal. Thus August Weismann’s view that all the rich diversity of animal life was merely the recombination of an unchanging hypothetical “idioplasm,” as well as more modern efforts to define evolution as the changing frequencies of genes in populations, also reflect the bias that qualitative constancy is more fundamental than change, that qualitative differences are in some sense illusory. Nevertheless, and grudgingly, science has accepted the reality of qualitative change and the importance of discontinuity. Phase transitions among the solid, liquid, and gaseous states are familiar examples. Continuous variation in the opposing forces that hold atoms together and pull them apart gives rise to thresholds at which the weaker force becomes the stronger, and the behavior of the whole is shifted.
Some qualitative changes in biology depend on such phase transitions in the underlying physical structures; for instance, enzymes denature at some critical temperature, and the waxy molecules of an insect’s cuticle lose their orientation when the temperature exceeds some threshold value, at which point insect’s body rapidly loses water. Other thresholds involve more complex interactions: the transitions from continuous development to dormancy in mammals take place with a shift in the length of day of less than half an hour. In each case structural and dynamic properties determine the threshold, but the particular molecules with these thresholds are present as a result of selection.
Underlying evolutionary change are two important kinds of qualitative change. First are changes in the characteristics of the organisms themselves, which may arise either abruptly or gradually as a consequence of continuous processes. But second, changes in the forces of selection can turn a side effect of some process into its main adaptive significance. Such changes can also turn a net disadvantage into an advantage; a characteristic that arose repeatedly in populations through nonadaptive processes but that was held to a minimum by selection suddenly is selected and sweeps through the population. Since most existing phenotypes are where they are because of a temporary balance of opposing forces, shifts in the context of selection can turn harmful or neutral traits into beneficial ones.
Moreover, every “trait”—every structure or physiological process in the organism—has many properties in addition to the ones that have been selected for in the course of its evolution. First there are properties that never interact with the environment, such as the color of an animal’s liver. Since it is dark inside, the color as such has absolutely no significance for the animal’s survival. The color is the consequence of various liver functions—breakdown of blood, production of digestive enzymes, localization of special biochemical processes. It is not a neutral trait, which might be expected to show random variation from species to species, nor is it an adaptive trait in its own right: it is the product of selection without having been selected for.
A similar phenomenon occurs in the rates of response of processes. The enzymes, which have been selected for their reaction rates at the temperatures to which the organism is normally exposed, all have reaction rates at temperatures never encountered in nature. These rates are not completely independent of the rates at normal temperatures. Since higher temperatures generally accelerate the rates of chemical reactions, organisms normally exposed to low temperatures often compensate by having highly reactive enzymes. But high reactivity at low temperature makes the enzymes so reactive at high temperatures that they become denatured easily and lose all activity. Therefore, the lower the temperature to which adaptation has taken place, the lower will be the denaturation temperature for the enzymes. That denaturation temperature is therefore an indicator of the circumstances of selection, even though certainly no enzymes have been selected to denature at 65° C.
The mammalian ear is obviously an organ of hearing, but it has other properties as well. For acoustic reasons it is a thin organ with a large surface area, the blood vessels cannot be deep, so heat is very readily lost. In fact, desert mammals often have extraordinarily large ears that serve as organs of temperature regulation. In this case a physical by-product of the evolution of an organ had properties that themselves became the objects of selection under the special conditions of the desert. But a large surface giving off heat, with circulation close to the surface, is very attractive to bloodsucking insects and ticks. And we observe that flies, mosquitoes, and ticks often congregate around the ears of their hosts. The adaptation of this organ both for hearing and heat dissipation creates a new problem, which is often met by the nervous and muscular pattern of ear twitching. Finally the contemporary ear is the result of a history of changing significance to the mammal.
The same thing happens at the population level. Population fluctuations, and in general the properties of population dynamics, depend on the demographic parameters of age-specific mortality and fecundity. In general, if reproduction is concentrated in a short period toward the end of life, the population will respond to perturbation by pronounced fluctuations. But if reproduction begins early and is spread over a wide time span, the fluctuations are much reduced. However, selection is acting on fecundity and mortality through their effect on the fitness of different genotypes in the population, not through their effect on population oscillations: Where environmental conditions such as high nonspecific mortality favor early reproduction, population fluctuations may be reduced; where there is an ecological advantage to delayed reproduction, fluctuations may be enhanced. But once a pattern of fluctuation has arisen, it is itself a fact of life for the species in question and for other species. Thus new selection pressures arise as a result of the previous evolution.
In summary: every characteristic has additional properties besides those initially selected for. These properties—the unselected consequences of selection—create both new possibilities and new vulnerabilities, and under altered circumstances these properties themselves can become the main object of selection. Furthermore, the evolutionary significance of a characteristic can change drastically from group to group or over time. The bones in the middle ear of mammals formed part of the jaw in our ancestors and further back had their origin in the gill arches of fishes. Regurgitation, the ability to eliminate irritating substances from the digestive tract, has been adapted to the feeding of the young in many groups and to defense in some gulls.
In the extreme case, the impossible becomes first possible and then necessary. The outstanding example of this is the oxygen revolution. Oxygen is a very toxic substance for most constituents of cells, and avoidance of or protection from oxygen must have had a very strong selective value at one time. Anaerobic organisms still survive in our world by living where oxygen does not penetrate. But some organisms dealt with oxygen by detoxifying it, allowing (indeed promoting) it to interact with some organic substances in the cell. This not only removed the oxygen as a poison, it also allowed the release of the chemical energy stored in those molecules, which increased metabolic efficiency drastically. Oxygen-using organisms eventually became overwhelmingly predominant among living things, but the dependence on oxygen itself created new vulnerabilities. Lack of oxygen is a more immediate threat to life than lack of food, so most organisms are excluded from oxygen-deficient habitats. Internal organs evolved to effectively distribute oxygen, and conditions that impede distribution—circulatory problems, anemia, carbon monoxide poisoning— are new threats to survival. On a smaller scale, certain microorganisms now not only tolerate but require the antibiotic streptomycin. And we can expect that some of the new toxic substances being introduced into our environment by uncontrolled industrial activity will someday become nutritional requirements of some bacteria.
Evolutionary ecology is not so much the study of changing characteristics of organisms in particular environments as the study of changing patterns of response to environment. But organisms do not respond to “the environment” as a whole; rather, they react to some aspect of the environment: an organism might detect the onset of winter by the shorter days, the lower temperatures, or the deteriorating nutrition. A predator may be detected by its silhouette or odor, a host plant by its shape, odor, or color.
Not all responses to the environment are adaptive. Sometimes trees, especially those that have been introduced into new climates, “misinterpret” warm weather in very early spring as indicating the end of the cold season; they begin to flower and form fruit, then lose their crop after a frost. Where a species has been exposed to a climatic pattern for a long time, selection acts on the norm of reaction in such a way as to reinforce those responses that improve survival. However, the survival value of a response is determined not by the physical properties of the factor responded to, but by what it indicates about those aspects of the environment that are critical for survival. The signal acts as a predictor of future conditions; how far in the future depends on the particular characteristics, the time it takes for a response to take place, and whether or not the response is reversible.
Signals that evoke behavioral responses are usually immediate indicators of food or danger, and the behavior itself disappears as it takes place. But some responses, such as dormancy, the change from vegetative growth to flowering, or conception in mammals, take longer. The signal evoking the response must indicate not only present but also future conditions. The vole responding to the condition of the grass by conceiving a smaller or larger litter is responding to an indicator of grass availability over the next five weeks of gestation and lactation; a tree responding to the onset of the rainy season may lose its leaves through desiccation if it responds prematurely to a drizzle, but risks defoliation if it delays long enough for the leaf-eating insects to emerge from their dry-season dormancy. Therefore the pattern of response to the environment is subject to intense selection that may be extremely local.
The adaptive system in animals consists of three parts. The first is the information-capturing system, which includes the familiar sense organs of animals. But it also includes special organs, such as the pineal eye of reptiles and the simple eyes of insects, which record not images but only the intensity and duration of light, and it includes more diffuse physiological states. The second part of the adaptive system is the response system, which includes the motor parts of the nervous system, the muscles, hormones that affect the mobilization of energy, hormones that regulate development and dormancy in insects and, at finer levels, the variable cellular components of biochemical regulation.
Both of these systems depend on the coordinated functioning of many strongly interacting components that mutually constrain each other. The relationship of parts, say in the visual system, does not depend on what is seen so much as on the properties of light; the coordination of muscles in flight or jumping is determined not by the reason for flight or jumping but on the mechanics of balance and locomotion. So all mammals are quite similar in their visual systems, and all respond physiologically to stress in similar ways: release of adrenalin and stored energy, increased blood pressure and heart rate, heightened alertness. What is different even between similar species is what constitutes stress, that is, how signals are interpreted. Thus the internal coherence of the information-capturing and response systems makes them rather conservative aspects of the organism. This conservatism often is misinterpreted to give an exaggerated notion of evolutionary continuity, especially in the discussion of human behavior.
The important differences lie in the system of signal interpretation that links the two systems. The interpretive system has several special properties. First, since the most advantageous response to a signal does not depend on the physical form of that signal but on its value as a predictor or correlate of other factors, different ecological contexts require different responses. Thus the interpretative system is highly labile. This results in the formation of local populations whose receiving and responding systems are similar but which differ in the thresholds and intensities of responses. For instance, insects that respond to short day length by entering a dormant state often show distinct latitudinal races; the length of day which indicates that another generation cannot be produced before winter varies with latitude. In contrast with the relative conservatism of the information-capturing and response systems, the coupling of signal to response is subject to rapid change and much variation.
Second, no two environmental situations are really identical, so it is not possible to have an adaptive system with separate rules of response for each circumstance. Rather, the organism ignores many differences in situations. As long as situations require the same response, their lumping imposes no disadvantage. But where similar circumstances require different responses, there is a great advantage in perceiving more subtle differentiations among environments, taking into account the co-occurrence of different kinds of signals entering through different pathways, and also taking into account the internal state of the organism, for example, its level of hunger. Therefore the interpretive system can become quite complex. A number of adaptive processes may use the same pathways, allowing the processes to influence each other in nonadaptive ways as well.
Third, if the response of an organism to its surroundings depends on the state of a single variable, say temperature, and if the appropriate response is to become active when temperature is between 14° C and 45° C, say, the response is clear-cut within that range. Ambiguity arises only around the threshold values 14° and 45°. But the more different factors that affect a response and the more alternative responses there are, the closer every real situation is to some threshold. Therefore, complex norms of reaction make ambivalence and ambiguity increasingly common, and whole new patterns of behavior may arise to resolve such ambiguities. The elaborate courtship rituals of many birds, fish, and mammals are procedures that distinguish members of the same species from other species, potential mates from competitors or prey. These behaviors can be seen as adaptations not to external environment but to the adaptive process itself.
Fourth, because the system that interprets environmental signals and determines the response is so complex, it must be described in terms of a large number of strongly interacting variables. Such a system is likely to be dynamically unstable, showing complicated fluctuations of state, and is unlikely to reach a resting state (stable equilibrium) even in the absence of all external signals. Thus spontaneous activity arises in organisms out of the complex evolution of responses to the environment. The central nervous system is a prime example: when isolated from external stimuli, it generates its own spontaneous patterns of activity. These new, internally generated activities may themselves have no initial adaptive significance although they are the results of adaptive evolution.
Finally, since the survival of the organism depends on the functioning of the system that interprets the environment, there is a great survival value in protecting that system both from external disruption and from internal breakdown. For instance, in mammals the brain has priority over other organs in the allocation of oxygen and energy.
The adaptive system is not limited to information capture, interpretation, and response. Many responses require some verification that the response has been completed. Sometimes this verification is directly accessible: an animal stops fleeing from a predator when it no longer detects the predator nearby. In other responses, however, the action itself takes only a short time, but its effect on survival or reproduction does not become manifest till much later. This is particularly the case for nutrition and reproduction. Starved animals do not feed continuously until they are in good health, nor do animals copulate until young appear. Some signal that is distinct from the adaptive value indicates that the response has been completed. Satiation of hunger and sexual release are correlates of nutrition and reproduction that serve as the direct feedback regulating the behavior. The appearance of these intermediate objectives of behavior is a consequence of the temporal disparity between cause and effect. But once they arise, these means become ends; whole complexes of behaviors have evolved around both feeding and sexuality that are no longer related to nutrition or reproduction.
The failure to understand qualitative change in evolution is especially pronounced in the study of human evolution. One form of biological determinism sees the origins of human behaviors in prehuman social behaviors and, emphasizing the continuity of evolution, assigns them the same significance. While conservative determinists look for a one-to-one correspondence between particular behaviors (for example, “aggressiveness” and human warfare), more flexible functionalists attempt to apply the rules of evolutionary ecology in a more general way. They argue that culture is the specifically human mode of adaptation to the environment and therefore that we can find the adaptive reasons for particular cultural practices.
This school provided a powerful antidote to the approach that saw cultural traits as essentially capricious. Harris (1974) and Vayda et al. (1960) argued that for Melanesians, raising pigs is a form of food storage and therefore is an adaptation to the uncertainty of crops. While cultural practices do have some ecological significance, this does not exhaust their social meaning: the processes of human adaptation introduce new phenomena. For instance, one buffer against local crop failure is the exchange of produce among localities. Therefore a functionalist may argue that exchange is an adaptation to environmental uncertainty. But with the evolution of exchange into trade, price intervenes to mediate, and price variation introduces more uncertainty into the food supply than drought. Similarly, the division of labor in production can be described in terms of technical efficiency, but in a class society it is also a way to organize exploitation. Or when the storage of food in the body is replaced first by external physical storage and then by the accumulation of wealth, credit, or obligations, there are no longer physical limits to reserves, and the possibility of insatiability arises.
The important point is that human society arises out of animal social organization, but as it arises, it transforms the significance of adaptations and creates new needs. As society gives rise to class divisions, the human population ceases to be the unit of adaptation. Thereafter, each regular interaction of people in a given culture with nature is determined by the interests of the different social classes in their conflictive or cooperative relations with each other.
The Origin of Life
Early evolutionists did not take up the problem of the origin of life as a central issue. In Origin of Species Darwin mentioned the problem only in passing and then metaphorically as the “primordial form, into which life was first breathed” (p. 484). Not only did the problem seem inaccessible, it veered dangerously close to theologized controversy. And separating the problem of the origin of a phenomenon from its subsequent trajectory seemed to fit into the Newtonian spirit of science.
During the latter part of the last century, microbiology was taking shape as a medically oriented science. Its greatest achievement—the germ theory of disease—was based on the discovery that even microbial life does not arise spontaneously but by invasion of a suitable medium by already formed microbes; spontaneous generation was exposed as myth. It was then argued, if life could arise from the nonliving in the past, why isn’t it happening now? A tentative answer was that the living arises very slowly from the nonliving, and that in a world teeming with life a new form would be gobbled up before it evolved very far. However, if the origin of life means the origin of organic molecules, a new problem arises: most biologically important chemical substances are readily oxidizable and would be burnt up in our atmosphere as fast as they formed. A world like ours, but without living things, is not a suitable place for life to arise. On the other hand, a world like ours with living organisms is equally unsuitable, because the primary requirement for the origin of life is the absence of life. Darwin (1871) understood this: “But if . . . we could conceive in some warm little pond, with all sorts of ammonia and phosphoric salts, light, heat, electricity, etc., present, that a protein compound was chemically formed ready to undergo still more complex changes, at the present day such matter would be instantly devoured or absorbed, which would not have been the case before living creatures were formed” (p, 18).
A major obstacle to the study of the origin of life was a philosophical bias that developed with the Copernican revolution and the Reformation: the view that people and, by extension, all forms of life, are insignificant. We were reminded that life occupies a film only a few dozen meters thick on the surface of a planet, that it would be virtually undetectable from space, and that it may be the product of a particular environment but not its cause.
A breakthrough came in the 1920s and 1930s as a result of discussions mostly among British and Soviet Marxists. Building on V, I. Vernadsky’s notion of the biosphere, and the earlier, more limited work of soil scientists who saw the soil as the joint product of physical and biological processes, they concluded that the present-day oxygen atmosphere is the product of life on earth and that the atmosphere within which life arose was quite different. Further, although life may have arisen in the sea, that sea was not like the present salt oceans, whose makeup is the result of the leaching of soils by the acids produced by organic decomposition. Thus, the problem was changed to one of co-evolution of the biosphere and its inhabitants.
Oparin (1957) embarked on a history of the chemical elements that make up living matter—mostly carbon, nitrogen, oxygen, hydrogen, sulphur, and phosphorus—first as free atoms in a stellar atmosphere, then in the simple compounds that were formed as the earth cooled. He concluded that the primitive earth had a reducing atmosphere of methane, ammonia, carbon dioxide, and water, and that under such conditions simple organic compounds would arise. Later experiments by Miller (1955) and others, using closed containers set up to resemble the primitive atmosphere and given energy in the form of electrical discharges, confirmed that complex mixtures of amino acids and other biologically important substances are formed in such an atmosphere. Different experiments give different mixtures, but the qualitative conclusion is that given almost any simulated primitive atmospheric environment, the formation of biologically important chemicals is virtually inevitable, and we are led to visualize a primitive sea as a thin soup of organic molecules.
Two schools exist on the question of the critical steps from organic soup to organisms. One view starts from the present-day universality of a genetic system based on nucleic acids (DNA) to argue that life originated in the gene, which then accumulated auxiliary structures around itself. The alternative is to consider that the gene itself was the product of a long evolution in primitive organisms. This view emphasizes that the components of DNA have other biologically important roles in cells and that their incorporation into a system of heredity presupposes the prior existence of auxiliary structures and processes.
But the origin of life requires more than the accumulation of organic molecules. First these molecules must be separated physically from the surrounding medium; this may have taken place on the surface of clays or through the formation of insoluble particles of a colloidal type. And the whole process has to be set in motion, since all living organisms are in dynamic flux, going through cycles of synthesis of proteins and nucleic acids, buildup of spatial organization, capture of energy and raw materials, and reproduction.
The question of the origin of life cycles, of dynamic systems out of a mixture of components, was unanswerable as long as process was seen as alien to matter. It was assumed that left to itself, a mixture of chemicals reaches some equilibrium state and nothing else happens unless some additional life principle is introduced from without. However, recent studies in the dynamics of complex systems suggest a different view: if we have an open system with many different kinds of components, and if even a small fraction of these accelerate or inhibit the formation of other components, then the system, instead of reaching some static equilibrium, has a good chance of being in constant flux, with concentrations of the various components rising and falling. Furthermore, in systems of only moderate complexity, the fluctuations can become regular, so that the system goes through repetitive cycles of activity.
The problem then becomes the regularization of fluctuation in living systems rather than the origin of change. Regularization could come about as follows. Many chemical substances, even simple ones, affect the rates of reactions in which they are not themselves permanently transformed; enzymes and coenzymes can increase reaction rates by many orders of magnitude. Further, these enzymes are highly specific, so that out of millions of possible interactions among the thousands of types of molecules, only thousands of reactions are accelerated. From the perspective of these accelerated reactions, the noncatalyzed chemical processes from an almost motionless background against which the critical biochemical processes take place. These are separated dynamically rather than isolated physically from the slower processes. Their fluctuations alter with changes of velocities and specificities mostly within this subsystem and can become regular cyclic processes. Thus the evolution of biological process is not the infusion of motion into a static system with all the necessary ingredients, but the modulation of chaotic motion, which is the natural state of existence for complex systems. This gives us some insight also into the processes of death: when enzymes cease to function, the high-velocity, specifically biological processes slow down and become submerged in the sea of background chemistry, and the system loses its kinetic identity.
Students of the origin of life are also concerned with the origin of the cell and with the great transformations of the biosphere that have accompanied biological evolution: the formation of the oxygen atmosphere, the emergence of soil as a geological-biological system, the creation of the protective ozone layer and of the oceans, the invasion of land by organisms. They also monitor the chemical revolution caused by human activity, which is introducing into the environment new molecular types at the rate of hundreds each year, as well as some familiar substances, such as carbon dioxide, at a rate that can alter atmospheric properties in a relatively short time. These changes, which introduce new selective forces that affect evolution, especially of microorganisms, may be comparable to the oxygen revolution in its long-term consequences.
Fitness of and in Populations
Changes in the genetic composition of populations by natural selection depend upon the relative reproductive rates of genotypes. A genotype that has a probability of .90 of living to adulthood and then producing two offspring has the same relative fitness as a genotype with a probability of survival of only .45 that will produce four offspring if it survives. There will be no change in the frequencies of these two genotypes in the population, because they are equally fit. But it makes a vast difference to a population whether it consists of individuals with high survivorship and low fertility or low survivorship and high fertility. The low-survivorship, high-fecundity population will use more food resources, spend more time and energy in parental care, occupy larger territories during breeding season, be more attractive to predators on juvenile forms, and so on. All these will have effects on the community of organisms in which the species lives. Whether a species develops such a pattern depends upon its interactions with other species in the community, even though forces internal to the species may drive it in the direction of that reproductive schedule.
Let us suppose that a mutation arises in a population of a food-limited species, which causes fecundity to double without changing the efficiency of food gathering and metabolism. The mutation will very quickly sweep through the population, which will then have a doubled fecundity. But because the species is food limited, the adult population will not be any larger than before. The newly evolved population will be better able to grow quickly if there is an increase in food supply, but its final numbers will not be greater than if it had lower fecundity. Moreover, if predators that specialize in eggs or juveniles switch their search image to this species with its more abundant young stages, the population may be reduced or even extinguished. The same deleterious consequences will occur if there are epidemic diseases whose propagation depends on crowding of the young. In general, the fact that a character has increased by natural selection within a population gives no information about the consequence of that evolution for the population or for the species as a whole. Evolutionary changes within a species may cause it to spread, to increase the number and size of its populations, or to become extinct. To understand the consequences, we need qualitative information about the biological change that has taken place and how it affects the relationship of the species to its resources and to other species.
Most species most of the time are roughly stable in geographical distribution and numbers, although both fluctuate. In the end, however, every species becomes extinct. No exact estimate is possible, but certainly fewer than one species in ten thousand that have ever existed survive. There were 280 genera of trilobites in thirty families at the beginning of the Ordovician, 500 million years ago, yet not a single trilobite was in existence 250 million years later at the end of the Permian. The average age of the carnivore genera now alive is only 8 million years, and the half-life for the carnivores known from the fossil record is only 5 million years. Extinction and speciation go on at roughly equal rates over broad groups, so the total number of species remains roughly constant although secular trends occur. Thus the number of families of bivalve molluscs has increased, slowly but steadily, by a factor of four in the last 500 million years because origination rates have been consistently somewhat higher than extinction rates, although both have fluctuated widely. Families of mammals, on the other hand, have declined over the last 30 million years by about 30 percent after rising by a factor of two in the previous 30 million, because the rates of appearance of new groups have fallen by a factor of five since the middle of the Oligocene. Some of these changes in extinction and origination rates can be rationalized by major geological events, such as the breakup of the single major continent Pangaea, beginning about 250 million years ago, or by climatic events of astronomical origin.
All such explanations are totally different in nature from Darwinian theory and do not even mention natural selection or genetics. They are framed in terms of changes in the diversity of habitats available or in climatic factors. Underlying the explanations are the assumptions that species will evolve to fill habitats or ecological niches as they become available, that species will become extinct as these niches disappear, and that climatic changes may occur at rates too fast for species to keep up. It is a view that makes a sharp division between organism and environment. The history of the environment in this view is driven by geological or astronomical forces, while organic evolution goes on in response to the opportunities created and destroyed by the history of the environment (see Chapter 2, on adaptation).
Organism and Environment
Preevolutionary biology stressed the harmony of nature, the correspondence of organism and environment, as evidence of the wisdom and benevolence of the creator. The environment was therefore seen mostly as resources, and the various structures of organisms as the means of obtaining these resources. This set as a research agenda the problem: what does this organism need for its development and where does this come from? For example, human beings require shade, shelter, and fuel, so forest trees were created to fulfill these needs.
The emergence of the theory of natural selection changed the attitude toward environment. More offspring are produced than can possibly survive, and the environment selects the more fit (or kills off the less fit). In the struggle for existence the environment is therefore seen as hostile—as stress, danger, obstacle. This role of the environment is expressed either as the passive absence of needed resources or as the active disruption of life processes (death through heat stress, infection, or predation). The research problem then becomes: how do organisms protect themselves against the environment? The scientist studies such problems as heat resistance, homeostasis, and adaptation. To these two aspects of ecology—environment as resource and environment as stress—modern work has added a third: environment as information.
The fundamental dichotomy of evolutionary theory is that of organism and environment. The organism is active, richly described, and changing; the environment is passive, delineated superficially, and treated as fixed in principle. Organisms are the proper objects of biological research, whereas environment is an auxiliary category falling within no present biological discipline. Some physical aspects of the environment, such as temperature, humidity, and insolation, as well as properties of soils, are of course studied by meteorology and climatology. (At the present time there is still no satisfactory biometeorology, that is, the characterization and analysis of the environment from the perspective of the organisms confronting it.) Some new environmental characterizations have been developed, such as evapotranspiration (the total water lost to the terrestrial ecosystem through evaporation from the ground and transpiration through the plant) and accumulated degree-days (insect species seem to complete their development when they have accumulated a certain number of degrees of temperature above a given threshold, and the measure of degree-days is used to predict emergence of major pests). But for the most part the description and analysis of environment in evolutionary studies is strikingly naive compared to the understanding of the structure and processes of organisms.
Some aspects of the environment must be mentioned before we can examine the organism-environment relation in greater detail. First, the environment is highly heterogeneous in time and space. The patterns of continuous variation of temperature or humidity shown on climatological maps represent averages, which obscure local gradients or discontinuities. Thus the vertical gradient in temperature from ground level up through the canopy even of low vegetation can be greater than 5°-10° C., with the greatest temporal variation at soil level. Horizontal differences between vegetated and bare spots, between soil and litter or bare rock can be even greater. The chemical environment within the soil, which is crucial for communities of microorganisms, often shows sharp changes over distances of one centimeter in the neighborhood of plant roots, and even small topographical differences between ridges and gulleys can be associated with drastic differences in the invertebrate populations. The heterogeneity of soil type is also discontinuous, and different types are often interspersed in complex patterns. Thus an ecologically meaningful characterization of the environment must give not only average conditions but also the variability in time and space and the “grain”—whether particular alternative conditions occur in big or small patches, or for long or short periods compared to the mobility or generation time of the organism under study.
The various aspects of the environment are not independent of each other. They tend, rather, to be associated in complexes, so an organism is not exposed to all possible combinations of temperature, humidity, day length, light intensity, and chemical conditions. This allows us to classify types of habitats and seasons. Because of the correlations among environmental conditions, organisms are able to use some conditions as indicators of others or as predictors of future conditions. Thus there arises the possibility that particular factors of the environment evoke responses that are not adaptations to those same factors but to conditions they indicate: the environment is met as information. The statistical pattern of the environment—the frequencies and durations of different conditions— defines the adaptive problems confronted by the organisms living in it and therefore their mode of evolution and the patterns of communities of species.
As a preliminary analysis, the separation of organism and environment or of physical and biological factors of the environment—of densitydependent or independent factors, of consumable or nonconsumable requirements—has proved useful. But it eventually becomes an obstacle to further understanding; the division of the world into mutually exclusive categories may be logically satisfying, but in scientific activity no nontrivial classifications seem to be really mutually exclusive. Eventually their interpenetration becomes a primary concern of further research. It is in this sense that dialectics rejects the doctrine of the excluded middle. Opposed to the model in which an organism is seen as inserted into an already given environment, we note several aspects of the organism-environment interpenetration.
Organisms select their environments. Animals do so actively, responding to environmental signals to find favorable habitats. Even over extremely short distances we find different populations: the upper and lower surfaces of leaves, sunflecks and shadows, gulleys and ridges often have quite different inhabitants. Rotten fruit set out as fly traps in various spots in an apparently homogeneous region will attract the various species in different proportions. And many animal species avoid the extreme stress of desert conditions by emerging only at dawn and twilight. Plants, which are of course less mobile, can orient their growth, coordinate dormancy with seasonal conditions, and evolve mechanisms of seed dispersal so that they are exposed to only part of the range of physical conditions of an area.
In general the selection of environments by organisms seems to be adaptive; habitat selection brings them in contact with more favorable conditions than would result from random movement. But it would be an oversimplification to interpret environment selection as the seeking of optimal conditions. First, what is optimal depends on the state of the organism, the result of its previous exposures to environment. Second, different processes in the organism may have different requirements, so the habitat selected may be a compromise among conflicting needs most adequately met in different places. (Many species reproduce, rest, and feed in different places.) The inhabitants of extreme conditions may not prefer or require the very high temperatures or salinities of their special habitats, but rather tolerate them to avoid predators or competitors. Finally, a habitat selected with respect to one aspect of the environment includes other environmental conditions that then become factors of selection.
Organisms modify their environments in several ways. They deplete the resources they consume; they excrete into the environment waste products they cannot use or that are harmful to them; and their presence in a habitat leaves evidence that attracts predators and parasites. These effects are byproducts of their activity and nonadaptive.
The structures and activities of organisms directly modify their immediate physical environment. At the surface of leaves of green plants is a film of air about a millimeter thick, which is richer in oxygen and moisture and poorer in carbon dioxide than the free atmosphere. For certain fungi and small insects, this surface film is their whole habitat; longer-legged leafhoppers’ bodies are above it. This boundary layer is both advantageous and disadvantageous to the plant. It retards water loss but also reduces evaporative cooling; it can reduce photosynthesis on clear, still days; it provides a habitat for algae and lichens, which may help the plant capture minerals from rainwater but also permits fungi and other pathogens to survive. The shape and texture of the leaf influence the persistence of this layer and are therefore subject to natural selection in response to opposing requirements.
Similarly, among the leaves of a plant there is often a region that is lower in temperature and light intensity and higher in humidity than the surrounding atmosphere. The layer of area around the skin of a mammal is warmer, wetter, and richer in urea and this is the environment to which mosquitoes have adapted their feeding behavior.
The physical influence of organisms on their environment extends further out as well. Trees usually modify wind and temperature conditions to a distance of about ten times their height, and they modify the soil conditions around their roots. A forest habitat is the product of all its plant, animal, and microbial inhabitants, which jointly stabilize the water regime, filter the light, reconstitute the soil organic matter, use up nutrients, add decomposition products, and regulate the weathering of the bedrock. If the forest is large enough, it also has some influence on rainfall and atmospheric conditions. Particular species act on their environments in special ways as well: earthworms, ants, termites, rodents, and peccaries move vast amounts of soil; the dry leaves of seasonal vegetation increase the frequency, extent, and intensity of fires; insects that defoliate large areas change the microclimate; wood-boring beetles and termites hollow out twigs, which ants use for nests but which also provide entrances for infection and thus change the life spans of trees.
Organisms transform the statistical structure of their environment. The state of the organism depends on certain weighted aspects of its environments in the past. Thus, for insects whose development depends on accumulated degree-days, the temperatures of the past are represented in the present as a sum. But an animal’s food gets used up, and the influence of previous feeding declines with time at a rate that depends on the biology of the species. For creatures with high metabolic rates, such as birds, the nutritional state is the food captured, over the last few days, say whereas for a scorpion it is the food intake over the last few weeks or months. Thus long-term averaging of the environment reduces its unpredictability. Further, the mobility of organisms within or between generations turns the spatial patchiness of the habitat into a temporal sequence of conditions. What faces short-lived or relatively immobile organisms as alternative environments may be met by more mobile or longer-lived species as averages of conditions over areas or years. And the felt heterogeneity of environment is significant only in relation to the organism’s tolerance for diverse conditions.
Organisms determine what aspects of their environment are relevant and which environmental variations can be lumped or ignored. For example, in bird communities the vertical pattern of vegetation density rather than the composition of plant species determines the suitability of the habitat, and the diversity in height of vegetation determines the diversity of bird species. But herbivorous insects in the same localities face the vegetation less as densities than as distributions of edible and inedible chemical compositions. For anoline lizards, whose food consists of insects, the resources are defined as a distribution of sizes of moving objects. For some species of ants such as Pheidole megacephala, which avoid very hot sunlight, a meadow may be a shifting patchwork of suitable and unsuitable environments, while for less demanding species the same place is a uniform foraging area.
As any environmental factor impinges on the organism, the physical form of the signal changes. Its effects spread out through many pathways within the organism; these pathways diverge and converge, and at each step the factor is represented by changes in activity or in amounts of substances. Thus in mammals, a fall in outside temperature results in increased consumption of sugar, changes in heart rate and circulation pattern, possible depletion of energy, and so on. Or a hotter environment, such that an insect can spend fewer hours a day searching for food, may be felt physiologically as hunger.
Organisms respond to their environments, Any aspect of the environment that impinges on the organism penetrates through multiple pathways. Temperature changes alter the rates of specific chemical reactions, act on sense receptors, may denature certain enzymes, stimulate particular neural activity, and evoke behavioral responses. We can trace the external influence through the organism. Some pathways enhance the signal: a small change in day length can make the difference between dormancy and continual development. Other pathways filter out external influences: wide fluctuations in food intake result in small differences in glucose available to the brain. Therefore the state of the organism over a range of environments is a combination of what happens to it and what it does.
Some features of the responses to the environment must be noted. The responses themselves seem to be the result of a tight network of mutually dependent interactions, while these as a cluster are more loosely bound to the processes that evoke the response. For example, the breaking of dormancy in plant seeds requires the softening or opening of the seed coat, the conversion of starch into available sugar, the initiation of root tip growth, and other processes, which are of necessity closely coordinated. But the signal that brings on these activities is quite flexibly connected to them and can differ even among closely related organisms. On the other hand, the structures and processes that capture environmental information may become linked to different responses. Thus related ant species use chemically similar alarm substances to signal danger, but one responds with defensive mobilization and the other with flight. We also note that animals are more similar in their fight-or-flight reactions to danger than in what constitutes danger. In the course of evolution, the different pathways can be enhanced or suppressed or recombined in so many ways that for environmental events which are not so extreme as to override the organism’s biological integrity, there is no necessary relation between the physical form of the signal and the response.
If organisms respond to their environments, then the environment may be read through the organism, and units of environmental measurement can be translated into units of phenotype. The Danish botanist Raunkiaer (1934) was the first to recognize this principle in his classification of life forms. Since the relative frequencies in a vegetation of trees, shrubs, herbs, vines, large and small leaves, entire and dissected leaves, evergreen and deciduous leaves, and thin and succulent leaves all reflect the climatic regime, a table giving such a distribution is also an indicator of the climate. A rain forest can be recognized by its life forms even when it is not raining.
The same principle applies to shorter-term environmental properties. For example, in the laboratory we can follow the growth of fruit flies at different temperatures and plot the number of bristles against temperature. Then we can collect flies in nature, find the average number of bristles, and find from the laboratory data the temperature at which those flies developed. Further, the variance among the wild flies, after correcting for the variance among flies raised under uniform conditions, indicates the variance among the inhabitants where the wild population developed.
The reciprocal interaction of organism and environment takes place through several pathways which link the individual and evolutionary time scales:
- Organisms actively select those environments in which they can survive and reproduce.
- For the individual this active selection determines what environmental impacts the organism will respond to. On the evolutionary scale it determines which environments the organism adapts to, what kind of selection it experiences.
- The environment acts differently on different genotypes. In some environments different genotypes may respond almost identically, while in others they may produce widely different phenotypes. In the environments commonly experienced by the population, there is less variation among the responses of different genotypes than in unusual or extreme environments. In moderately extreme environments, the differences among genotypes are amplified, but in very severe environments genetic differences become irrelevant; uniformity returns as lethality. Therefore the environment as developmental stimulus helps turn genetic variability into available phenotypic variability, which environment as Darwinian filter selects. Much evolutionary theory ignores this double effect of environment.
- The way in which the organism modifies the environment depends on its genotype. Some environmental impacts enhance survival more than others. Therefore the environment selects the pattern of its own modification.
Every part or activity of an organism acts as environment for other parts. Much of evolution is the adaptation of parts of the organism to each other. Most of the arguments about organism-environment interaction apply to the organism’s internal environment as well.
“Environment” cannot be understood merely as surroundings, no matter how dynamically. It is also way of life; the activity of the organism sets the stage for its own evolution. This strong interaction between what an organism does and what happens to it is especially dramatic in human evolution. Engels’ essay fragment, “The Role of Labor in the Transition from Ape to Man,” drafted sometime between 1872 and 1882, explores this relation in the Lamarckian framework of direct inheritance of acquired characters. But if we replace that direct causation by the action of natural selection, the critical argument remains valid: the labor process by which the human ancestors modified natural objects to make them suitable for human use was itself the unique feature of the way of life that directed selection on the hand, larynx, and brain in a positive feedback that transformed the species, its environment, and its mode of interaction with nature.
Integration of Parts
Hegel warned that the organism is made up of arms, legs, head, and trunk only as it passes under the knife of the anatomist. Physiology and embryology have filled in many of the details of the intricate interdependence of the parts of the body; interdependence permits survival when the parts function well, but in pathological conditions, it produces pervasive disaster.
The correlation among parts is also seen in systematics, in that most conceivable combinations of traits never occur. For instance, there are not small grass-eating reptiles or flying molluscs. On the other hand, in the course of evolution the relations among parts do change: D’Arcy Thompson (1917) showed that if animal shapes are drawn in outline on a rubber sheet that is then stretched in various ways, corresponding to changes in relative growth rates in different directions, we can produce the shapes of other related species. Therefore much evolution can be interpreted as the uncoupling of relative growth rates. Gould (1977) has argued that the relative dissociability of somatic from sexual maturation accounts for the frequently observed phenomena that are misinterpreted as recapitulation: neoteny (the deceleration of somatic relative to sexual development) and progenesis (the attainment of sexual maturity in juvenile and even larval bodies). When we trace the evolution of particular lines, we find that rapid evolution in some traits leaves others unchanged. Indeed, it is these conservative characters that permit the tracing of the phylogeny.
Therefore the problem is how to deal with the intimate integration and relative dissociability of parts of the organism in the same theoretical framework. We must combine developmental and adaptive arguments in this analysis. Different parts of the organism may be correlated for various reasons. Their development may respond to a common stimulus. For instance, in mammals the steroid hormones that promote growth are also involved in sexual maturation and in the development of the secondary sexual characteristics. Also, traits that develop independently may have a common inhibitor. This mechanism is important in insects, in which the juvenile hormone suppresses many otherwise independent developmental processes. Or one structure may directly induce the other, as in the induction of development of the eye by the neural optic cup that lies beneath it.
Two parts of the organism may mutually regulate each other’s growth. For instance, both the minerals absorbed by the roots of a plant and the carbohydrates synthesized in the leaves are required for the growth of roots and leaves. But each structure has first access to its own product. If the leaves are partly removed, new growth will be mostly above ground, while if roots are pruned, root growth is accelerated, reestablishing a stable rootshoot ratio. The particular ratio maintained is genetically determined and can be altered by grafting shoots of one genotype onto roots of another.
Similar tissues may take up similar nutrients and hormones and give off similar products, so if such tissues are near each other they are in a sense competing for resources. Anything that alters the partitioning of resources between them generates a negative correlation, while factors that affect the concentrations of nutrients, hormones, waste products, or physical conditions around them induce a positive correlation.
A single gene may affect characters that are seemingly remote from each other. This phenomenon of pleiotropy reflects the process of gene action: genes cause the production of enzymes, which catalyze particular chemical reactions in the complex metabolic network. A genetic change that alters the rate of a reaction or even blocks it completely will have effects that spread out through the network.
Early stages of development may affect later ones; for instance, in mammals infant size (as affected by nutrition) influences adult size. Although all of these correlations are the direct consequences of the processes of development, they are not rigidly determined. The rates of flow of substances between parts, the sensitivities of different tissues to the same stimulus, the timing of the growth periods of different organs, and the rates of production or breakdown of growth-promoting or inhibiting substances in different tissues are all subject to selection and may be changed. Some effects of a gene may be enhanced while others are reduced as a result of a selection regime. Similarly, under extreme environmental conditions the regulatory system may break down and the correlation of parts drastically altered.
Therefore we have to inquire, what are the selection pressures operating on the integration of parts? First, some parts function together mechanically. Thus the proper occlusion (fit of upper and lower teeth) is more important than the absolute size of the teeth. The ball and socket fit of long bones at joints, the ratios of bone lengths as levers in running, the positioning of parts for balance, the fit of skull to brain and integument to internal organs all have obvious survival value.
Second, some parts that are physically quite different function together. An alteration in the size and structure of the limbs associated with altered feeding or escape behavior requires correlated changes in bones, muscles, innervation, and circulation. Mutual regulation among these parts allows such adaptation to occur relatively rapidly.
Third, different organs or processes that have little direct interaction may be bound together ecologically by their common adaptive significance. For example, most termites are nocturnal foragers. They have little tolerance for dry heat and are very vulnerable and attractive to predators. Any species switching over to daytime foraging would require an increase in desiccation resistance and in protection against predators (perhaps through the formation of a soldier caste). Thus, physiological tolerance, diurnal habit, and defense capacity are ecological correlates.
Finally, some parts of an organism function to maintain other parts within satisfactory limits. Thus constancy of body temperature is the result of coordinated variability in heart action, circulation, metabolic rate, activity of sweat glands, and voluntary activity.
Various aspects of an organism may be bound together as “traits” if they are units of either development or selection. But if the direction of selection is altered, they may lose their cohesion and evolve independently. For instance, corn breeders are now interested in improving the nutritional value of the crop. Nutritional value for humans is certainly not a natural trait of Zea mays. But when the breeders combine total yield, percentage of protein, proportion of lysine in the protein, and so on into a selection value, a number of biochemical properties have been linked as a unit of evolution, an adaptive trait. Should the breeding program be abandoned, the “trait” itself would lose evolutionary meaning, and its parts would be dispersed among other components of survival.
The same applies to behavioral traits. Because of contemporary social and political concern with “violence” and “aggression,” some students of behavior have begun to treat “aggressiveness” as a trait. When they define measures of aggressiveness (the number of times a white rat bites the research assistant) and select animals for high indices of aggressiveness, they have in fact created the trait as a unit of evolution. “Trait”ness is a property not of the index itself but of the circumstances of its development and selection. Such experiments do not demonstrate the reality of hereditary aggressiveness in other species or even in other populations of rats.
So far we have emphasized the developmental reasons for the association of parts and the ecological reasons for the retaining of that association. But the significance of the components of an ecological cluster of structures and processes is not limited to their role in that cluster. Circulation in a limb is certainly related to the use of that limb, but it is also related to heat balance and to competing needs for blood (as in digestion). Furthermore, the separate, internally tightly integrated ecological characteristics may be only loosely coupled to each other. For instance, the mouth parts of a sucking insect are related to the physical structure of its food source, and its dormancy pattern is related to the seasonality of its habitat. Therefore, it is possible for one pattern to change without the other changing.
We visualize the organism as a system of variables grouped in overlapping clusters of components of fitness (survival and reproduction) and subject to constraints of physical limitation and developmental interdependence. A changed environment can result in selection on several of these clusters. In general, among the important variables of a cluster, the least constrained will change the most; often these are the traits that appear late in development and locally.
Three aspects of adaptation counter the advantages of tighter integration. First, if a particular characteristic is subject to different selection pressures, and if the optimal states of the characteristic under the two pressures separately are not too different, then the outcome is likely to be a compromise in which the part in question is determined by both aspects of fitness (Levins 1968). But as the directions of selection pressures diverge, compromise becomes less possible. Finally, one pressure swamps the other, and the characteristic evolves under the control of only one adaptive criterion. Or a part may subdivide: if the optimum tooth shape for tearing is so different from the optimum shape for grinding that an intermediate tooth shape is disastrous for both functions, the control over front and back teeth may separate, producing a mixed strategy of incisors and molars. Or if the digestion of different foods requires different levels of acidity, instead of having some intermediate pH that allows only slow digestion, we have an alkaline mouth and an acidic stomach.
A second, related phenomenon that favors uncoupling is that as the number of interacting variables and the intensity of their interaction increases, it becomes increasingly difficult for selection to increase fitness. Species with very tight coupling are unable to adapt as readily as those in which different fitness components are more autonomous. Finally, the more strongly coupled and interdependent the system, the more pervasive the breakdown when some stress overwhelms the regulatory capacity. Therefore, what has taken place in evolution is the successive coupling and uncoupling of parts as the advantages of coordinated functioning and mutual regulation oppose the disadvantages of excessive constraint and vulnerability. There is no general rule as to which is better. Among the most abundant organisms are mammals, with tight integration, and plants, which have greater autonomy of parts.
Macroscopic and Microscopic Theories
There are really two theories of evolution, microscopic and macroscopic, by analogy to microscopic and macroscopic physics. Quantum mechanics is not really relevant to the laws of the movement of falling bodies except insofar as the existence of coherent macroscopic physical objects and their interaction with the physical medium in which they move are reflections of their microscopic properties. In the same way, the particular changes that occur when a new mutation is incorporated into a species may increase or decrease the species’ probability of survival, depending upon the unique biological interactions involved, but explanations of general patterns of diversity in space and time must be framed in terms of phenomena at a different level. Each instance of speciation or extinction is a consequence of microscopic events that are ultimately dependent upon the genetic composition of the species, which has been molded by microevolutionary processes. The two theories can never make effective contact until the concept of relative fitness of genotypes within a population is connected to the fitness of populations and species in ecological communities. But this connection cannot be made until the dichotomy of organism and environment is broken down. The divorce between the relative fitness of genotypes and the fitness of populations arises from the fiction that new varieties are selected in a fixed environment, so that the only issue is whether, given that environment, they will produce fewer or more offspring. But in reality, a new variety means a new environment, a new set of relations among organisms and with inorganic nature. On the other hand, each mutational change cannot result in a totally new relation between organism and environment, or else no cumulative evolutionary change could ever take place.
Over and over again, terrestrial vertebrates have adapted to aquatic life by developing finlike appendages from their terrestrial organs. This has occurred independently in whales, seals, penguins, and even in sea snakes, which are laterally flattened. If every small change in morphology led to a radical change in predators or food resources, the evolution of such obviously adapted swimming forms could never occur. We must assume that the relations between phenotype and fitness has at least two general properties. First, there must be continuity, so that very small changes in morphology, physiology, and behavior usually have only a small effect on the ecological relations of the organism. Continuous deformations of phenotype should map frequently into continuous deformations of ecological relation. Second, characters must be quasi-independent. That is, there must exist a large number of possible phenotypic correlations between a given character change and other aspects of the phenotype. If character correlations are unbreakable, or nearly so, then no single aspect of the phenotype, like fins, could ever develop without totally altering the rest of the organism in generally nonadaptive ways. At the same time, despite the principle of continuity, there are points at which quantitative change becomes qualitative, and the principle of quasi-independence does not mean that every kind of restructuring of organisms is possible. These two principles are the beginning of a theory of the evolution of organisms. The theory still must be developed; at the moment we have only a kinematics of the evolution of abstract genotypes.
2 Adaptation
This chapter was first published as “Adattamento” in Enciclopedia Einaudi, vol. 1, edited by Giulo Einaudi (Turin, Italy, 1977). The present text is the English original, which was translated into Italian for the encyclopedia.
EVERY theory of the world that is at all powerful and covers a large domain of phenomena carries immanent within itself its own caricature. If it is to give a satisfactory explanation of a wide range of events in the world in a wide variety of circumstances, a theory necessarily must contain some logically very powerful element that is flexible enough to be applicable in so many situations. Yet the very logical power of such a system is also its greatest weakness, for a theory that can explain everything explains nothing. It ceases to be a theory of the contingent world and becomes instead a vacuous metaphysic that generates not only all possible worlds, but all conceivable ones. The narrow line that separates a genuinely fruitful and powerful theory from its sterile caricature is crossed over and over again by vulgarizers who seize upon the powerful explanatory element and, by using it indiscriminately, destroy its usefulness. In doing so, however, they reveal underlying weaknesses in the theories themselves, which can lead to their reformulation.
This element of immanent caricature is certainly present in three theoretical structures that have had immense effects on twentieth-century bourgeois thought: Marxism, Freudianism, and Darwinism. Marx’s historical materialism has been caricatured by the vulgar economismi that attempts to explain the smallest detail of human history as a direct consequence of economic forces. Freud’s ideas of sublimation, transference, reversal, and repression have been interpreted to explain any form of overt behavior as a direct or transformed manifestation of any arbitrary psychological cause. In Darwinism the element that is both central to the evolutionary world view and yet so powerful that it can destroy Darwinism as a testable theory is adaptation.
The concept of adaptation not only characterizes explanations of the evolution of life forms but also appears in cultural theory as functionalism. According to the concept there exist certain “problems” to be “solved” by organisms and by societies; the actual forms of biological and social organizations in the world are seen as “solutions” to these “problems.” Describing adaptation in these modern terms should not mask the fact that the concept has been inherited from a much older world view, one that was characteristic of the aristocratic and fixed world before the European bourgeois revolution. In that view the entire universe, including living organisms and especially the human species and its social organization, was perfectly fitted to serve a higher purpose. “The heavens declare the glory of God and the firmament showeth his handiwork” are the words of the Psalmist. The universe was the work of a divine creator, and its parts were made by him to fit together in a harmonious way, each part subserving the higher function. In the view of some, the primary object of this creation was man, whose nature was carefully fashioned to allow a new and more trustworthy race of angels to develop. The rest of the living world was designed to serve humankind. Cows were ideally designed to provide people with milk, and trees to give shade and shelter. The most important political consequence of this world view was the legitimation it provided for social organization. Lords and serfs, masters and slaves represented a division of power and labor that was necessary for the proper functioning of society and the working out of the divine plan.
The belief that organisms were marvelously fitted to their environments and that each part of an organism was exquisitely adjusted to serve a special function in the body, just as parts of the body politic were perfectly fitted to serve the needs of “society,” was carried over into modern biological and anthropological thought. All that changed was the explanation. Having rejected the supreme designer as responsible for the world’s perfection, Darwin needed to show that evolution by natural selection could lead to the same end. “In considering the origin of species, it is quite conceivable that a naturalist . . . might come to the conclusion that each species . . . had descended, like varieties, from other species. Nevertheless, such a conclusion, even if well founded, would be unsatisfactory until it could be shown how the innumerable species inhabiting this world have been modified, so as to acquire that perfection of structure and coadaptation which most justly excites our admiration” (Darwin 1859, p. 3). Indeed, in his chapter “Difficulties of the Theory,” Darwin realized that “organs of extreme perfection and complication” were a critical test case for his theory. “To suppose that the eye, with all its inimitable contrivances for adjusting the focus to different distances, for admitting different amounts of light, and for the correction of spherical and chromatic aberration, could have been formed by natural selection seems, I freely confess, absurd in the highest degree” (p. 186). But such “organs of perfection” are only the extreme and obvious results of the process of natural selection, which lies at the center of Darwinian evolutionary theory. For Darwin, species originated through a continuing process of adaptation which, at the same that it produced new species, produced organisms whose parts were in harmony with each other so that the organism as a whole was in harmony with its environment.
Being adapted and becoming adapted
The concept of adaptation implies that there is a preexistent form, problem, or ideal to which organisms are fitted by a dynamical process. The process is adaptation and the end result is the state of being adapted. Thus a key may be adapted to fit a lock by cutting and filing it, or a part made for one model of a machine may be used in a different model by using an adaptor to alter its shape. There cannot be adaptation without the ideal model according to which the adaptation is taking place. Thus the very notion of adaptation inevitably carried over into modern biology the theological view of a preformed physical world to which organisms were fitted. When the world was explained as the product of a divine will, there was no difficulty with such a concept, since according to the creation myth the physical world was produced first and the organisms were then made to fit into that world. The Divine Artificer created both the physical world and the organisms that populated it, so the problems to be solved and the solutions were products of the same schema. God posed the problems and gave the answers. He made the oceans and gave fish fins to swim in them, he made the air and put wings on birds to fly in it. Having created the locks, il Alto Fattore made the keys to fit them.
With the advent of evolutionary explanations, however, serious problems arose for the concept of adaptation. Certainly the physical universe predated living organisms, but what are the physical schemata to which organisms are adapting and adapted? Are there really preexistent “problems” to which the evolution of organisms provides “solutions”? This led to the concept of ecological niche. The niche is a multidimensional description of all the relations entered into by an organism with the surrounding world. What kind of food, and in what quantities, does the organism eat? What is its pattern of spatial movement? Where does it reproduce? At what times of day and during what seasons is it active? To maintain that organisms adapt to the environment is to maintain that such ecological niches exist in the absence of organisms and that evolution consists in filling these empty and preexistent niches.
But the external world can be divided up in an uncountable infinity of ways, so there is an uncountable infinity of conceivable ecological niches. Unless there is a preferred or correct way in which to partition the world, the idea of an ecological niche without an organism filling it loses all meaning. The alternative is that ecological niches are defined only by the organisms living in them, but this raises serious difficulties for the concept of adaptation. Adaptation cannot be a process of gradual fitting of an organism to the environment if the specific environmental configuration, the ecological niche, does not already exist. If organisms define their own niches, then all species are already adapted, and evolution cannot be seen as the process of becoming adapted.
Indeed, even if we put aside ecological niches, there are difficulties in seeing evolution as a process of adaptation. All extant species, for a very large part of their evolutionary histories, have neither increased nor decreased in numbers and range. If a species increased on the average by even a small fraction of a percent per generation, it would soon fill the world and crowd out all other organisms. Conversely, if a species decreased on the average, it would soon go extinct. Thus for long periods of its evolutionary lifetime, a species is adapted in the sense that it makes a living and replaces itself. At the same time, the species is evolving, changing its morphology, physiology, and behavior. The problem is how a species can be at all times both adapting and adapted.
A solution to the paradox has been that the environment is constantly decaying with respect to the existing organisms, so the organisms must evolve to maintain their state of adaptation. Evolutionary adaptation is then an infinitesimal process in which the organism tracks the ever-changing environment, always lagging slightly behind, always adapting to the most recent environment, but always at the mercy of further historical change. Both the occasional sudden increases in abundance and range of a species and the inevitable extinction of all species can be explained in this way. If the environment should change in such a way that the present physiology and behavior of a species by chance makes it reproductively very successful, it may spread very rapidly. This is the situation of species that have colonized a new continent, as, for example, the rabbit in Australia, finding there by sheer chance environmental conditions (including the lack of competitors) to which it is better adapted than it had been to its native habitat. Eventually, of course, such a species either uses up some resource that had existed in great excess of its needs or otherwise alters the environment by its own activity so that it is no longer able to increase in numbers. The alternative, that the environment remains unchanged but that the species by chance acquires a character that enables it to utilize a previously untapped resource, is very much less likely. Such favorable mutations, or “hopeful monsters” may nevertheless have occurred as, for example, in the evolution of fungus gardening by ants.
The simple view that the external environment changes by some dynamic of its own and is tracked by the organisms takes no account of the effect organisms have on the environment. The activity of all living forms transforms the external world in ways that both promote and inhibit the life of organisms. Nest building, trail and boundary marking, the creation of entire habitats, as in the dam building of beavers, all increase the possibilities of life for their creators. On the other hand, the universal character of organisms is that their increase in numbers is self-limited, because they use up food and space resources. In this way the environment is a product of the organism, just as the organism is a product of the environment. The organism adapts the environment in the short term to its own needs, as, for example, by nest building, but in the long term the organism must adapt to an environment that is changing, partly through the organism’s own activity, in ways that are distinctive to the species.
In human evolution the usual relationship between organism and environment has become virtually reversed in adaptation. Cultural invention has replaced genetic change as the effective source of variation. Consciousness allows people to analyze and make deliberate alterations, so adaptation of environment to organism has become the dominant mode. Beginning with the usual relation, in which slow genetic adaptation to an almost independently changing environment was dominant, the line leading to Homo sapiens passed to a stage where conscious activity made adaptation of the environment to the organism’s needs an integral part of the biological evolution of the species. As Engels (1880) observed in “The Part Played by Labor in the Transition from Ape to Man,” the human hand is as much a product of human labor as it is an instrument of that labor. Finally the human species passed to the stage where adaptation of the environment to the organism has come to be completely dominant, marking off Homo sapiens from all other life. It is this phenomenon, rather than any lucky change in the external world, that is responsible for the rapid expansion of the human species in historical time.
Extinction may be seen as the failure of adaptation in that genetic or plastic changes in an adapted species are unable to keep up with a change in the environment. A species’ response to environmental alteration is limited by the morphological, physiological, and behavioral plasticity given by its present biology and by genetic changes that may occur by mutations and natural selection. Phenotypic and genetic plasticity is thus limited in kind but, more important, it is limited in rate of response, so the environment is sure eventually to alter in a way and at a rate that outdistances the species’ adaptive response. More than 99.9 percent of all species that ever existed are extinct, and all are sure to be extinguished eventually.
The theory of environmental tracking does not solve the problem of evolution. It cannot explain, for example, the immense diversification of organisms that has occurred. If evolution is only the successive modification of species to keep up with a constantly changing environment, then it is difficult to see how the land came to be populated from the water and the air from the land, or why homoiotherms (warm-blooded organisms) evolved at the same time that poikilotherms (cold-blooded organisms) were abundant. This evolutionary diversification cannot be described in any consistent way as a process of adaptation unless we can describe preferred ways of dividing up the multidimensional niche space toward which species were evolving and, therefore, adapting. That is, the concept of adaptation is informative only if it has some predictive power. It must be possible to construct a priori ecological niches before organisms are known to occupy them and then to describe the evolution of organisms toward these niches as adaptation.
The exploration of other planets does provide the possibility of making such predictions, yet it also illustrates the epistemological difficulties involved. If there really are preexistent niches to which organisms adapt, then it ought to be possible to predict the kind of organisms (if any) that will be discovered on Mars or Venus, by examining the physical environments of those planets. In the building of devices to detect life on these planets, predictions are in fact being made, since the detection depends upon the growth of hypothetical organisms in defined nutrient solutions. These solutions, however, are based on the physiology of terrestrial microorganisms, so the devices will detect only those extraterrestrial life forms that conform to the ecological niches already defined on earth. If life on other planets has partitioned the environment in ways that are radically different from those on earth, those living forms will remain unrecorded. There is no way to use adaptation as the central principle of evolution without recourse to a predetermination of the states of nature to which this adaptation occurs, yet there seems no way to choose these states of nature except by reference to already existing organisms.
Specific adaptations
Evolutionists, having accepted that evolution is a process of adaptation, regard each aspect of an organism’s morphology, physiology, and behavior as a specific adaptation, subserving the state of total adaptation of the entire organism. Thus fins are an adaptation for swimming, wings for flying, and legs for walking. Just as the notion of adaptation as an organism’s state of being requires a predetermined ecological niche, so, even more clearly, assigning the adaptive significance of an organ or behavior pattern presumes that a problem exists to which the character is a solution. Fins, wings, and legs are the organism’s solutions to the problem of locomotion in three different media. Such a view amounts to constructing a description of the external environment and a description of the organism in such a way that they can be mapped into each other by statements about function.
In practice the construction may begin with either environment or organism, and the functional statement then used to construct the corresponding structure in the other domain. That is, the problems may be enumerated and then the organism partitioned into solutions, or a particular trait of an organism may be assumed to be a solution and the problem reconstructed from it. For example, the correct mutual recognition of males and females of the same species is regarded as a problem, since the failure to make this identification would result in the wastage of gametes and energy in a fruitless attempt to produce viable offspring from an interspecific mating. A variety of characters of organisms, such as color markings, temporal patterns of activity, vocalizations (as in the “mating call” of frogs), courtship rituals, and odors, can then be explained as specific adaptations for solving this universal problem. Conversely, the large erect bony plates along the middorsal line of the dinosaur Stegosaurus constitute a character that demands adaptive explanation; they have been variously proposed as a solution to the problem of defense, either by actually interfering with a predator’s attack or by making the animal appear larger in profile, as a solution to the problem of recognition in courtship, and as a solution to the problem of temperature regulation by acting as cooling fins.
Hidden in adaptive analyses are a number of assumptions that go back to theistic views of nature and to a naive Cartesianism. First it must be assumed that the partitioning of organisms into traits and the partitioning of environment into problems has a real basis and is not simply the reification of intuitive human categories. In what natural sense is a fin, leg, or wing an individual trait whose evolution can be understood in terms of the particular problem it solves? If the leg is a trait, is each part of the leg also a trait? At what level of subdivision do the boundaries no longer correspond to “natural” divisions? Perhaps the topology as a whole is incorrect. For example, the ordinary physical divisions of the brain correspond in a very rough way to the localization of some central nervous functions, but the memory of events appears to be diffusely stored, and particular memories are not found in particular microscopic regions.
As we move from anatomical features to descriptions of behavior, the danger of reification becomes greater. Animal behavior is described by categories such as aggression, altruism, parental investment, warfare, slave making, and cooperation, and each of these “organs of behavior” is provided with an adaptive explanation by finding the problem to which it is a solution (Wilson 1975). Alternatively, the problems to be solved in adaptation also may be arbitrary reifications. For example, by extension from human behavior in some societies, other animals are said to have to cope with “parent-offspring conflict,” which arises because parents and offspring are not genetically identical but both are motivated by natural selection to spread their genes (Trivers 1974). A whole variety of manifest behaviors, such as the pattern of parental feeding of offspring, is explained in this way. Thus, the noise-making of immature birds or humans is a device to coerce the selfish parents into feeding their offspring, who otherwise would go untended.
A second hidden assumption is that characters can be isolated in an adaptive analysis; any interactions among characters are considered to be secondary and to represent constraints on the adaptation of each character separately. Similarly, each environmental problem to be solved is isolated and its solution regarded as independent of other interactions with the environment, which are at most constraints on the solution. Obviously, a ceteris paribus argument is necessary for adaptive reconstructions; otherwise all traits would have to be considered in the solution to all problems and vice versa, leading to a kind of complex systems analysis of the whole organism in its total environment, The entire trend of adaptive evolutionary arguments is toward a Cartesian analysis into separate parts, each with its separate function.
The third hidden assumption is that all aspects of an organism are adaptive. The methodological program of adaptive explanation demands an a priori commitment to such explanations for all traits that can be described. This commitment establishes the problematic of the science as one of finding the adaptation, not of asking whether it exists at all. The problematic is an inheritance from the concept of the world as having been designed by a rational creator so that all aspects of it have a function and can be rationalized. The problem of explanation is to reveal the workings of this rational system.
The weakness of evolutionary theory is manifest in the assumption that all traits, arbitrarily described, are adaptive. If the assumption is allowed to stand, then adaptive explanations simply become a test of the ingenuity of theorists and of the tolerance of intellectuals for tortured and absurd stories. Again, it is in behavioral traits that the greatest scope for rationalization appears, for example, explanations of the supposed mass suicide of lemmings by drowning as being a population regulation device that is adaptive for the species as a whole. If, on the other hand, the assumption is dropped, traits that are difficult to rationalize can be declared nonadaptive, allowing evolutionists to explain just those traits that seem most obviously to fit their mode of explanation, relegating the others to the category of “non-Darwinian” (King and Jukes 1969). Some evolutionists (Kimura and Ohta 1971) now regard a large part of the variation in protein structure among species as random, irrational, and non-Darwinian, but this is bitterly contested by conventional Darwinians who accept that adaptationist methodological program without reserve (Ford 1975).
Given the assumptions of the adaptationist program, there are great difficulties and ambiguities in determining the adaptation of a given organ. Every trait is involved in a variety of functions, yet it cannot be claimed to be adaptation for all. Thus a whale’s flipper can destroy a small whaling boat, but no one would argue that the flipper is an adaptation for destroying surface predators rather than for swimming. Nor does the habitual and “natural” use of an organ necessarily imply that it is an adaptation for that purpose. The green turtle, Chelonia mylas, uses its front flippers to propel itself over dry sand to an egg-laying site above high-water mark, then digs a deep hole for the eggs in a slow and clumsy way, using its hind flippers as a trowel. But the turtles use these swimming paddles in this way for lack of anything better; flippers cannot be regarded as adaptations either to land locomotion or to hole digging. If sufficiency of an organ is not a sufficient condition of its being an adaptation, neither is necessity of an organ a necessary condition. Every terrestrial animal above the size of an insect must have lungs, because the passive transpiration of gases across the skin or by a tracheal system would not suffice for respiration in a large volume. Lungs can properly be considered an adaptation for breathing because without them the animal would suffocate, but most adaptations are not so essential. The striping of zebras may be an adaptation to protective camouflage in tall grass, but it is by no means certain that a species of unstriped zebras would go extinct from predation, or even that they would be less numerous.
The problem of judging the adaptive importance of a trait from its use becomes more difficult when the use itself must be reconstructed. The bony plates of Stegosaurus may have been a device for temperature regulation, predator protection, and species recognition simultaneously. Nor is this doubt restricted to extinct forms. Some modern lizards have erectile “sails” along their dorsal lines and or brightly colored, inflatable gular pouches. These may serve as both aggressive display and sexual recognition signals, and the dorsal spines may also be heat regulators. In principle, experiments can be done on living lizards to determine the effect of removing or altering these characters, but in practice the interpretation of such alterations is dangerous, since it is not clear whether the alteration has introduced an extraneous variable. Even if it could be shown that an organ functions in a variety of ways, the question of its adaptation is not settled because of the implied historical causation in the theory of adaptation. The judgment of whether the lizard’s gular pouch is an adaptation for species recognition depends upon whether natural selection is supposed to have operated through the more frequent correct matings of individuals with the pouch. If, when the pouch reached a certain size, it also incidentally frightened predators, it would be a preadaptation for this latter purpose. The distinction between those uses for which an organ or trait is an adaptation and those for which it is a preadaptation could be made only on historical grounds by a reconstruction of the actual forces of natural selection. Even for extant organisms, this is impossible.
In the absence of actual historical data on natural selection, the argument that a trait is an adaptation rests on an analysis of the organism as a machine for solving postulated problems. Using principles of engineering, the investigator performs a design analysis and compares the characteristics of the postulated design with those of the organ in question. Thus the postulate that the dorsal plates of Stegosaurus are adaptations for heat exchange rests on the porous nature of the bone, suggesting a large amount of blood circulation; on the larger size of the plates over the most massive part of the body, where heat production is greatest; on the alternating unpaired arrangement of the plates to the left and right of the midline, suggesting the proper placement of cooling fins; and on the constriction of the plates at their base, nearest the heat source, where they would be inefficient radiators. A more quantitative engineering analysis is sometimes made, proposing that the organ or character is actually optimal for its postulated purpose. Thus Leigh (1971), using hydrodynamic principles, showed that the shape of a sponge is the optimal shape for that creature, on the supposition that the problem for the sponge is to process the maximum amount of food-containing water per unit time.
The fit is not always perfect, however. Orians (1976) has calculated the optimal distribution of food sizes for a bird that must search for and catch prey, then return with it to a nest (central-place foraging). A comparison of the prey caught with the distribution of available prey sizes did indeed show that birds do not take food items at random, that they are biased toward larger items; however, they do not behave according to the calculated optimum. The explanation offered for the failure of a close fit is that because of the competing demand to visit the nest often enough to discourage predators, the birds spend less time searching for optimal prey than they would if the behavior were a pure adaptation to feeding efficiency. This is a paradigm for adaptive reconstruction. The problem is originally posed as efficiency of food gathering. A deviation of the behavior from random in the direction predicted is regarded as strong support for the adaptive explanation, and the discrepancy from the predicted optimum is accounted for by an ad hoc secondary problem that acts as a constraint on the solution to the first. There is no methodological rule that instructs the theorist in how far the observation must deviate from the prediction before the original adaptive explanation is abandoned altogether. By allowing the theorist to postulate various combinations of problems to which manifest traits are optimal solutions, the adaptationist program makes of adaptation a metaphysical postulate that not only cannot be refuted but is necessarily confirmed by every observation. This is the caricature that was immanent in Darwin’s insight that evolution is the product of natural selection.
Natural selection and adaptation
A sufficient mechanism for evolution by natural selection is contained in three propositions:
- There is variation in morphological, physiological, and behavioral traits among members of a species (the principle of variation).
- The variation is in part heritable, so that individuals resemble their relations more than they resemble unrelated individuals and, in particular, offspring resemble their parents (the principle of heredity).
- Different variants leave different numbers of offspring either in immediate or remote generations (the principle of differential fitness).
It is important to note that all three conditions are necessary as well as sufficient conditions for evolution by natural selection. If the variants do not differ in their reproductive success, then of course there is no natural selection. The existence of heritable variation is especially crucial. If variation exists but is not passed from parent to offspring, then the differential reproductive success of different forms is irrelevant, since all forms will produce the same distribution of types in the next generation. Any trait for which the three principles apply may be expected to evolve. That is, the frequency of different variant forms in the species will change, although it does not follow in all cases that one form of the trait will displace all others. There may be stable intermediate equilibria at which two or more variant forms coexist at a characteristic stationary frequency.
These necessary and sufficient principles for evolution by natural selection contain no reference to adaptation. Darwin added the postulate of adaptation to explain the mechanical cause of the phenomenon of differential reproduction and survival. The “struggle for existence,” according to Darwin, was the result of the tendency of species to reproduce in excess of the resources available to them, an idea he got from reading Malthus’s (1798) Essay on the Principle of Population. The struggle would be won by those individuals whose morphology, physiology, and behavior allowed them to appropriate a greater share of the resources in short supply, or those who could survive and reproduce on a lower resource level, or those who could utilize a resource that was unsuitable for their competitors. In these latter two forms the struggle for existence was freed from the idea of actual struggle between individuals. “I should premise that I use the term Struggle for Existence in a large and metaphorical sense . . . Two canine animals in a time of dearth may be truly said to struggle with each other which shall get food and live. But a plant at the edge of the desert is said to struggle for life against the drought” (Darwin 1859, p. 62).
Given this struggle in its “large and metaphorical sense,” an engineering analysis should be able to predict which of two individuals will better survive and reproduce. By studying the bones and muscles of the legs of two zebras and by applying simple mechanical principles, one should be able to say which of the two can run faster and therefore better escape predators. Further, it is in principle possible to predict the direction of evolution of leg muscles and bones by a local differential analysis, since the superior of any two slightly different shapes can be discerned.
The struggle for existence also redirects the idea of adaptation from an absolute to a relative criterion. So long as organisms are considered only in relation to their ecological niche, they are either adapted, in which case they will persist, or they are unadapted and are on their way to extinction. But if individuals of the same species are considered in relation to each other, they are competing for the same set of resources or struggling to reproduce in the same unfavorable environment (the plants at the edge of the desert), and their relative adaptation becomes the focus. Two forms of a species might both be absolutely adapted in the sense that the species would persist if it were made up entirely of either form, yet when placed in competition the greater adaptation of one would lead to the extinction of the other. By the same consideration, the relative adaptation of two distinct species cannot in general be compared because species are never competing with each other in the same exclusionary way as are forms of the same species. If two species overlapped so much in their ecological niches that their abundances were critically determined by the same limiting resource, one species would become extinct in the competition. Occasionally, of course, an introduced species does extinguish another species, as in the case of the Mediterranean fruit fly, which was extinguished in eastern Australia by the sudden southward spread of the Queensland fruit fly, a very close relative that lays its eggs in the same cultivated fruit. At first sight, the engineering approach to differential fitness seems to remove the apparent tautology in the theory of natural selection. Without this design analysis Darwinian theory would simply state that the more fit individuals leave more offspring in future generations and would then determine relative fitness from the number of offspring left by different individuals. Since, in a finite world of contingent events, some individuals will, even by chance, leave more offspring than others, there will be a posteriori tautological differences in fitness among individuals. From that, one can only say that evolution occurs because evolution occurs. The design analysis, however, makes it possible to determine fitness a priori, and therefore one can judge the relative adaptation of two forms in the absence of any prior knowledge of their reproductive performances.
Or can one? The conditions for predicting from relative adaptation analysis are the same as for judging absolute adaptation. A change in length of the long bones of zebras’ legs, allowing them to run faster, will be favored in evolution provided (1) that running speed is really the problem to be solved by the zebra, (2) that the change in speed does not have countervailing adverse effects on the animal’s adaptation to solving other problems set by the environment, and (3) that lengthening the bone does not produce countervailing direct developmental or physiological effects on other organs or on its own function. Even though lions prey on zebras, it is not necessarily true that faster zebras will escape more easily, since it is by no means certain that lions are limited by speed in their ability to catch prey. Moreover, greater speed may be at the expense of metabolic efficiency, so if zebras are food limited, the problem of feeding may be made worse by solving the problem of escaping from predators. Finally, longer shank bones may be more easily broken, cost more developmental energy to produce, and create a whole series of problems of integrated morphology. Relative adaptation, like the judgment of absolute adaptation, must be a ceteris paribus argument and, since all other things are never equal, the final judgment as to whether a particular change in a trait will produce relatively greater adaptation depends upon the net effect on the entire organism. The alternative would be to maintain that the engineering analysis of a predetermined problem is to be taken as defining the adaptation, irrespective of its net benefit to the organism. Such a solution would decouple adaptation from evolution and make it into a purely intellectual game.
Evolutionary convergence
The serious methodological and epistemological difficulties in the use of adaptive explanations should not blind us to the fact that many features of organisms clearly seem to be convergent solutions to obvious environmental problems. It surely is no accident that fish have fins; that aquatic mammals have altered their appendages to form finlike flippers; that ducks, geese, and seabirds have webbed feet; that penguins have paddlelike wings; and even that sea snakes, lacking fins, are flattened in cross- section. All these traits are obviously adaptations for aquatic locomotion, and the reproductive fitness of the ancestors of these forms must have been increased by the gradual modification of their appendages in a similar way. Yet it seems pure mysticism to suppose that swimming was a major “problem” held out before the eyes of the terrestrial ancestors of all these animals before they actually had to cope with locomotion through a liquid medium, It must be that the problem of swimming was posed in a rudimentary and marginal form, putting only marginal demands on an organism, whose minor adaptive response resulted in a yet deeper commitment of the evolving species to the water.
But this coevolution of the organism and of the environment it was creating for itself continued over long times in the same direction, producing fishlike animals from doglike ones and swimmers from fliers, all with flattened appendages. It follows that the ceteris paribus argument must be true reasonably often, or else no progressive alteration to form such structures could occur. Therefore, the mapping of character states into net reproductive fitness must have two characteristics: continuity and quasiindependence. By continuity we mean that very small changes in a character result in very small changes in the ecological relations of the organism and therefore very small changes in reproductive fitness. Neighborhoods in character space map into neighborhoods in fitness space. So a very slight change in the shape of a mammalian appendage to make it finlike does not cause a dramatic change in the sexual recognition pattern or make the organism attractive to a completely new set of predators. By quasi-independence we mean that there exists a large variety of paths by which a given character may change; although some of these paths may give rise to countervailing changes in other organs and in other aspects of the ecological relations of the organism, in a reasonable proportion of cases the countervailing effects will not be of sufficient magnitude to overcome the increase in fitness from the adaptation. In genetic terms, quasiindependence means that a variety of mutations may occur, all with the same effect on the primary character but with different effects on other characters, and that some set of these changes will not be at a net disadvantage.
Nonadaptive characters and the failure of adaptation
While the principles of continuity and quasi-independence can be used to explain adaptive trends in characters that have actually occurred, they cannot be used indiscriminately to assert that all characters are adaptive or to predict the appearance of some character that ought to evolve because it would be adaptive. The lack of continuity and quasi-independence may, in fact, be powerful deterrents to adaptive trends. That adaptation has occurred seems obvious. But it is not at all clear that most changes, or even many, are adaptive. The adaptationist program is so much a part of the vulgarization of Darwinism that an increasing amount of evolutionary theory consists in the uncritical application of the program to both manifest and postulated traits of organisms.
A paradigm is the argument by Wilson (1975) that indoctrinability (“human beings are absurdly easy to indoctrinate . . . they seek it”, p. 562) and blind faith (“men would rather believe than know,” p. 561) are adaptive consequences of human evolution since conformist individuals will more often submit to the common goals of the group, guaranteeing support rather than hostility and thus increasing their reproductive fitness. This view universalizes two socially determined behaviors, makes them part of “human nature,” and then argues for their adaptive evolution. Putting aside the question of the universality of indoctrinability and blind faith, the claim that they are the product of adaptive evolution requires that there has been heritable variation for these traits in human evolutionary biology, that conformists really would leave more offspring, all other things being equal and, finally, that all other things are equal. None of these propositions can be tested. There is no evidence of any present genetic variation for conformism, but that is not compelling since the question concerns genetic variation in the evolutionary past. Nor is there any reason to suppose that conformism is a separate trait and not simply a culturally defined concept that has been reified by the biologist. The alternative is to recognize that “conformism” is a “trait” only by abstract construction, that it is one of the possible ways of describing some aspect of the behavior of some individuals at some times and that it is a consequence of the evolution of a complex central nervous system. That is, the adaptive trait is the extremely highly developed central nervous organization; the appearance of conformity as a manifestation of that complexity is entirely epiphenomenal.
A parallel situation for morphological characters has long been recognized in the phenomenon of allometry. Different organs grow at different rates, so that if growth is prolonged to produce a larger individual not all parts are proportionately larger. For example, in primates tooth size increases less from species to species than does body size, so large primates have proportionately smaller teeth than small primates. This relationship of tooth size to body size is constant across all primates, and it would be erroneous to argue that for some special adaptive reason gorillas have been selected for relatively small teeth. Developmental correlations tend to be quite conservative in evolution, and many so-called adaptive trends turn out on closer examination to be purely allometric.
Reciprocally, the increase of certain traits in a population by natural selection is not in inself a guide to adaptation. A mutation that doubled the egg-laying rate in an insect, limited by the amount of food available to the immature stages, would very rapidly spread through the population. Yet the end result would be a population with the same adult density as before but twice the density of early immatures and much greater competition among larval stages. Periodic severe shortages of food would make the probability of extinction of the population greater than it was when larval competition was less. Moreover, predators may switch their search images to the larvae of this species now that they are more abundant, and epidemic diseases may more easily spread. It would be difficult to say precisely what environmental problem the increase in fecundity was a solution to.
Adaptation as ideology
The caricature of Darwinian adaptation that sees all characteristics, real or constructed, as optimal solutions to problems has more in common with the ideology of the sixteenth century than with that of the nineteenth. Before the rising power and eventual victory of the bourgeoisie, the state and the unchanging world were seen and justified as manifestations of divine will. The relations among people, and between humankind and nature, were unchangeably just and rational because the author of all things was unchanging and supremely just and rational. There was, moreover, an organic unity of relationships, for example, of lord and serf and of both to the land, which could not be broken, since they were all part of an articulated plan. This ideology, which was both a conscious legitimation of the social order and its unconscious product, necessarily came under attack by the ideologues of the increasingly powerful commercial bourgeoisie. The success of commercial and manufacturing interests made it necessary for men to be able to rise as high in status and power as their entrepreneurial activities took them and required freeing money, land, and labor power from their traditional rigid relationships. It had to be possible to alienate land for primary production and by the same process to allow the laborer to own his own labor power and to carry it to the centers of manufacturing where he could sell it in the labor market. Thus the ideology of the Enlightenment emphasized progress rather than stasis, becoming rather than being, and the freedom and disarticulation of parts of the world, rather than their indissoluble unity. Voltaire’s Dr. Pangloss, who believed that even the death of thousands in the Lisbon earthquake proved that this was the “meilleur des mondes possibles,” symbolized the foolishness of the old ideology. Descartes’ bete machine and La Mettrie’s homme machine provided the program for the analysis of nature by dissecting and disarticulating it into separate causes and effects.
Darwin’s work came at the end of the successful struggle of the bourgeoisie to make a world appropriate to its own activities. The middle of the nineteenth century was a time of immense expansion of production and wealth. Darwin’s maternal grandfather, Josiah Wedgwood, started as a potter’s apprentice and became one of the great Midland industrialists, epitomizing the flowering of an exuberant capitalism. Mechanical invention and a free labor market underlay the required growth of capital and the social and physical transformation of Europe. Herbert Spencer’s Progress: Its Law and Cause, expressed the mid-nineteenth-century belief in the inevitability of change and progress. Darwin’s theory of the evolution of organic life was an expression of these same ideological elements. It emphasized that change and instability were characteristic of the living world (and of the inorganic world as well, since the earth itself was being built up and broken down by geological processes). Adaptation, for Darwin, was a process of becoming rather than a state of final optimality. Progress through successive improvement of mechanical relations was the characteristic of evolution in this scheme.
It must be remembered that for Darwin, the existence of “organs of extreme perfection and complication” was a difficulty for his theory, not a proof of it. He called attention to the numerous rudimentary and imperfect forms of these organs that were present in living species. The idea that the analysis of living forms would show them, in general, to have optimal characters would have been quite foreign to Darwin. A demonstration of universal optimality could only have been a blow against his progressivist theory and a return to ideas of special creation. At the end of Origin of Species (1859) he wrote: “When I view all beings not as special creations, but as lineal descendants of some few beings which lived long before the first bed of the Cambrian system was deposited, they seem to me to become ennobled. Judging from the past, we may safely infer that not one living species will transmit its unaltered likeness to a distant futurity . . . And as natural selection works solely by and for the good of each being, all corporeal and mental endowments will tend to progress toward perfection “ (p. 489).
Even as Darwin wrote, however, a “spectre was haunting Europe.” The successful revolutions of the eighteenth century were in danger of being overturned by newer revolutions. The resistance by the now dominant bourgeoisie to yet further social progress required a change in the legitimating ideology. Now it was claimed by their advocates that the rise of the middle classes had indeed been progressive but that it was also the last progressive change; liberal democratic entrepreneurial man was the highest form of civilization, toward which the development of society had been tending all along. Dr. Pangloss was right after all, only a bit premature. The liberal social theory of the last part of the nineteenth century and of the twentieth has emphasized dynamic equilibrium and optimality. Individuals may rise and fall in the social system, but the system itself is seen as stable and as close to perfect as any system can be. It is efficient, just, and productive of the greatest good for the greatest number. At the same time the Cartesian mechanical analysis by disarticulation of parts and separation of causes has been maintained from the earlier world view.
The ideology of equilibrium and dynamic stability characterizes modern evolutionary theory as much as it does bourgeois economics and political theory; Whig history is mimicked by Whig biology. The modern adaptationist program, with its attempt to demonstrate that organisms are at or near their expected optima, leads to the consequence that although species come into existence and go extinct, nothing really new is happening in evolution. In contrast to Darwin, modern adaptationists regard the existence of optimal structures, perfect adaptation, as the evidence of evolution by natural selection. There is no progress because there is nothing to improve. Natural selection simply keeps the species from falling too far behind the constant but slow changes in the environment. There is a striking similarity between this view of evolution and the claim that modern market society is the most rational organization possible, that although individuals may rise or fall in the social hierarchy on their individual merits, there is a dynamic equilibrium of social classes, and that technological and social change occur only insofar as they are needed to keep up with a decaying environment.
3 The Organism as the Subject and Object of Evolution
This chapter was first published in Scientia 118 (1983): 63-82.
THE MODERN theory of evolution is justly called the “Darwinian” theory, not because Darwin invented the idea of evolution, which he certainly did not, nor because Darwin’s invention, natural selection, is the only force in evolution. Rather, Darwin realized that the process of evolutionary change of living organisms is radically different from any other known historical process and because his formulation of that process was a radical epistemological break with past theories. Before Darwin, theories of historical change were all transformational. That is, systems were seen as undergoing change in time because each element in the system underwent an individual transformation during its life history. Lamarck’s theory of evolution was transformational in regarding species as changing because each individual organism within the species underwent the same change. Through inner will and striving, an organism would change its nature, and that change in nature would be transmitted to its offspring. If the necks of giraffes became longer over time, it was because each giraffe attempted to stretch its neck to reach the top of the trees. An example of a transformational theory in modern natural science is that of the evolution of the cosmos. The ensemble of stars is evolving because every star, after its birth in the initial explosion that produced the matter of the universe, has
undergone the same life history, passing into the main sequence, becoming a red giant, then a white dwarf, and finally burning out. The evolution of the universe is the evolution of every star within it. All theories of human history are transformational; each culture is transformed through successive stages, usually, it is supposed, by transformation of the individual human beings that make up the society.
In contrast, Darwin proposed a variational principle, that individual members of the ensemble differ from each other in some properties and that the system evolves by changes in the proportions of the different types. There is a sorting-out process in which some variant types persist while others disappear, so the nature of the ensemble as a whole changes without any successive changes in the individual members. Thus variation among objects in space is transformed qualitatively into temporal variation. A dynamic process in time arises as the consequence of a static variation in space. There is no historical process other than the evolution of living organisms that has this variational form, at least as far as we know.
In transformational theories the individual elements are the subjects of the evolutionary process; change in the elements themselves produces the evolution. These subjects change because of forces that are entirely internal to them; the change is a kind of unfolding of stages that are immanent in them. The elements “develop,” and indeed the word “development” originally meant an unfolding or unrolling of a predetermined pattern, a meaning it still retains in photography and geometry. The role of the external world in such developmental theories is restricted to an initial triggering to set the process in motion. Even Lamarck’s theory of organic evolution did not make the environment the creator of change but only the impetus for the organism to change itself through will and striving. Two characteristics flow from such a transformational view. First, the stages through which each individual passes are themselves the precondition for the next stage. There are no shortcuts possible, no reordering of the transformation, and only one possible end to the process. Indeed, the tensions and contradictions of one stage are actually the motive forces of the change to the next stage. Marx’s theory of history is precisely such a theory of well-ordered historical stages, each of which gives rise to the next as a consequence of forces internal to each step. Theories of psychic development, such as those of Freud and Piaget, are derived from theories of embryological development of the nineteenth century. Each stage, whether of the body or of the psyche, is a necessary precondition of the next stage and leads to it because of forces that are purely internal at each moment. The role of the outer world is to set the process in motion and to allow the successful completion of each step.
This role of the environment provides the second characteristic of transformational theories, the possibility of arrested development. If external forces block the unrolling, the system may become permanently fixed at an early stage, and it is this premature fixation that explains any observed variation from individual to individual. In Freudian theory the personality may become fixed at an anal or oral erotic stage or at the stage of Oedipal resolution and so give rise to the manifest variations among neurotic symptoms.
In the theory of neoteny, evolutionary theory retains notions of linear arrays of stages and arrested development. According to this view organisms that appear later in evolution have the form of earlier developmental stages of their ancestral species. Gorilla and human embryos resemble each other much more than the adults do, and adult humans are morphologically like the gorilla fetus. Humans are thus gorillas born too soon and fixed at a gorilla fetal stage. It follows from such a theory that if the development of a human being could somehow be unblocked, it would develop the long arms, receding jaw, and sagittal crest of the adult gorilla that is present but hidden. It seem obvious that a neotenic view of evolution is severely limited in its scope, since adult humans cannot be said to resemble the early embryonic stages of fish. Indeed, evolution cannot be any kind of simple unfolding, for such a homunculus theory implies the absurdity that mammals are already completely contained in the earliest single-celled organisms.
Darwin’s variational theory is a theory of the organism as the object, not the subject, of evolutionary forces. Variation among organisms arises as a consequence of internal forces that are autonomous and alienated from the organism as a whole. The organism is the object of these internal forces, which operate independently of its functional needs or of its relations to the outer world. That is what is meant by mutations being “random.” It is not that mutations are uncaused or outside of a deterministic world (except as quantum uncertainty may enter into the actual process of molecular change), but that the forces governing the nature of new variations operate without influence from the organism or its milieu. Once variation has occurred, some variants survive and reproduce while others are lost to the species, according to the relation between the variant types and the environment in which they live. Once again the organism is the object, this time of external forces, which are again autonomous and alienated from the organism as a whole. The environment changes as a consequence of cosmological, geological, and meteorological events that have their own laws, independent of the life and death of the species. Even when the environment of a given species includes other species, the histories of those species are autonomous and independent of the species being considered.
The roles of the external and the internal are not symmetrical in Darwinism. Pre-Darwinian variational theories placed the internal forces of development in the dominant position and understood history as a consequence of development. Neoteny belongs to this Platonic, preDarwinian tradition for it portrays the evolution of organisms as nothing but various stages of arrested development; ontogeny dominates history. In Darwinian theory the reverse is true. Historical forces are dominant, and development does nothing but provide the raw material for the forces of natural selection. The external chooses which of many possible internal states shall survive. Thus the developmental pathways that we see are the consequence of history, not its cause. Ernst Haeckel’s theory of recapitulation is, in this sense, truly Darwinian, for it holds that the embryonic stages through which an organism passes are the trace of its evolutionary past, not the image of its evolutionary future. Human embryos have gill slits because their fish and amphibian ancestors had them, but in human beings the gill slits disappear because human beings have evolved further. Through evolution, new stages of development have been added, stages that were not immanent in Devonian fish. So history in Darwinism dominates ontogeny.
Thus classical Darwinism places the organism at the nexus of internal and external forces, each of which has its own laws, independent of each other and of the organism that is their creation. In a curious way the organism, the object of these forces, becomes irrelevant for the evolutionist, because the evolution of organisms is only a transformation of the evolution of the environment. The organism is merely the medium by which the external forces of the environment confront the internal forces that produce variation. It is not surprising, then, that some vulgar Darwinists make the gene the only real unit of selection and see evolution as a process of differential survival of genes in response to the external world. In The Selfish Gene Richard Dawkins (1976) speaks of organisms as “robots . . . controlled body and mind” by the genes, as nothing but a gene’s way of making another gene. If the species is indeed the passive nexus of gene and selective environment, if the genes propose and the environment disposes, then in a deep sense organisms really are irrelevant, and the study of evolution is nothing but a combination of molecular biology and geology.
But such a view gives a false picture of organic evolution and cannot successfully cope with the problems posed by evolutionary biology, for it ignores two fundamental properties of living organisms that are in direct contradiction to a superficial Darwinism. First, it is not true that the development of an individual organism is an unfolding or unrolling of an internal program. At a symposium in 1982 commemorating the hundredth anniversary of Darwin’s death, a leading molecular biologist expressed the belief that if the complete sequence of an organism’s DNA were known and a large enough computer were available, it would be possible, in principle, to compute the organism. But that is surely false, because an organism does not compute itself from its DNA. The organism is the consequence of a historical process that goes on from the moment of conception until the moment of death; at every moment gene, environment, chance, and the organism as a whole are all participating. Second, it is not true that the life and death and reproduction of an organism are a consequence of the way in which the living being is acted upon by an autonomous environment. Natural selection is not a consequence of how well the organism solves a set of fixed problems posed by the environment; on the contrary, the environment and the organism actively codetermine each other. The internal and the external factors, genes and environment, act upon each other through the medium of the organism. Just as the organism is the nexus of internal and external factors, it is also the locus of their interaction. The organism cannot be regarded as simply the passive object of autonomous internal and external forces; it is also the subject of its own evolution.
Gene and development
It is common, even in textbooks of genetics, to speak of genes determining traits, as if knowing the gene means the trait of the organism is given. This notion derives from several historical sources. First, since the nineteenth century, embryologists have taken their problematic to be explaining how a fertilized egg of a frog always becomes a frog, while that of a chicken always develops into another chicken. Even when the environment in which development is taking place is severely disturbed, a process of regulation often assures that the final outcome is the same. If the developing limb bud of an amphibian embryo is cut out, the cells disaggregated, then put back together again, and the lump of cells reimplanted in the embryo, a normal leg will develop. And no environmental disturbance has ever caused an amphibian embryo to develop into a chicken. Thus there is an overwhelming impression that a program internal to the cells is being expressed and that the development of the adult is indeed the unfolding of an inevitable consequence.
Second, the laws of inheritance were discovered by following simple traits that have a one-to-one correspondence to genes. Mendel succeeded where others had failed partly because he worked with horticultural varieties in which major differences in phenotype resulted from alternative alleles for single genes. Mendel’s peas had a single gene difference between tall and short plants, but in the usual natural populations of most plant species there is no simple relation at all between height and genes. So when Mendel tried to understand the inheritance of variation in the wild species Hieracium, he failed completely.
Third, modern molecular biology deals with the direct products of gene action, the proteins produced by the cell using specific sequence information from the structure of DNA. As with Mendel’s peas, there is a one-to-one correspondence between a simple genetic difference and a discrete observable difference in phenotype. Indeed, the problematic of molecular biology is to give a complete description of the machinery that is responsible for assembling the unique correspondence. It is impossible to work out the details of the machinery if the correspondence between gene and phenotype is poor, so molecular biology, by the necessary demands of its research methods, concentrates all its attention on the simplest relations between gene and trait. If, however, one examines the more general relations between gene and organism, it becomes immediately apparent that the situation is more complex.
In general, the morphology, physiology, metabolism, and behavior—that is, the phenotype—of an organism at any moment in its life is a product both of the genes transmitted from the parents and of the environment in which development has occurred up until that moment. The number of light-receptor cells, or facets, in the compound eye of the fruit fly, Drosophila, is usually about 1,000, but certain gene mutations severely reduce the number of facets. For example, flies carrying the mutation Ultrabar have only about 100. However, the number of eye cells also depends upon the temperature at which the flies develop; flies of the normal genotype produce about 1,100 cells at 15°C, but only 750 cells at 30°C.
The relationship between the phenotype and the environment is expressed in the norm of reaction, which is a table or graph of correspondence between phenotypic outcome of development and the environment in which the development took place. Each genotype has its own norm of reaction, specifying how the developing organism will respond to various environments. In general, a genotype cannot be characterized by a unique phenotype. In some cases the norm of reaction of one genotype is consistently below that of another in all environments. So, for example, we can say unambiguously that Ultrabar flies have smaller eyes than normal flies because that is true at every temperature of development. However, another mutation, Infrabar, also has fewer cells than normal, but it has an opposite relation to temperature, and its norm of reaction crosses that of Ultrabar (see Fig. 3.1). Clearly we cannot ask, “Which mutation has more eye cells?” because the answer depends upon temperature. Fig. 3.2 shows the reaction norms for the probability of survival of immature stages in Drosophila as a function of temperature. The different lines represent genotypes taken from a natural population, and they are more typical of norms of reaction than are the mutations of eye size. There is no regularity at all to be observed. Some genotypes decrease survival with temperature, some increase it, some have a maximum at intermediate temperatures, some a minimum. The genotype 2', which has the third highest survival at 14°C, has the lowest at 26°C. The experiments illustrated in Figs. 3.1 and 3.2, carried out in a variety of organisms for a variety of traits and a variety of environments, establish three characteristics for the relationship among gene, environment, and organism. First, there is no unique phenotype corresponding to a genotype; the phenotype depends on both genotype and environment. Second, the form and direction of the environment’s effect upon development differs from genotype to genotype.
Third, and reciprocally, there is no unique ordering of genotypes such that one can always be characterized as “superior” or “inferior” to another.
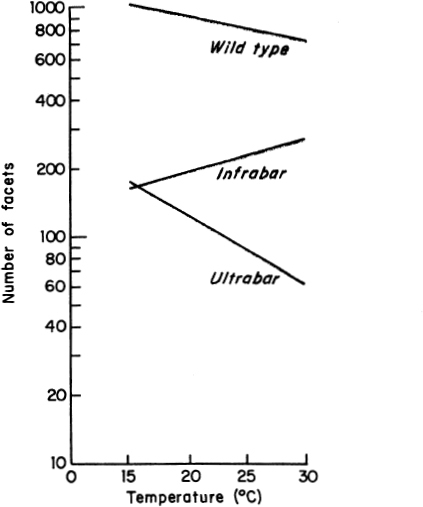
Fig. 3.1. Reaction norms for the number of eye cells as a function of temperature in Drosophila.
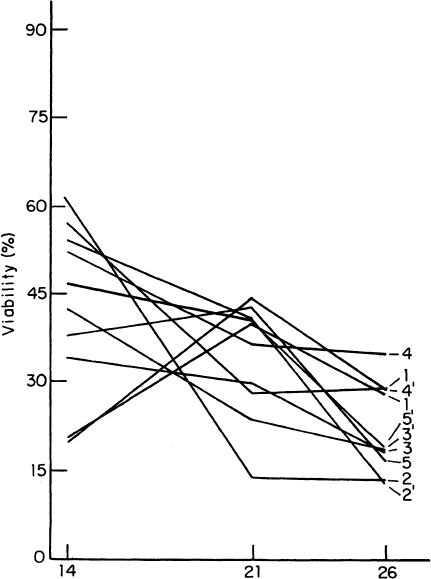
Fig. 3.2. Reaction norms for viability in genotypes from natural populations of Drosophila, as a function of temperature.
While the phenotype depends on both genotype and environment, it is not determined by them. If one counts the eye cells or the large hairs on the left and right side of a Drosophila raised in the laboratory, one finds that the fly is usually asymmetrical but that there are as many right-sided flies as left-sided. That is, there is no average difference between left and right for the species as a whole, but there is a large variation among individuals. The genes of the left and right side of a fly are the same. Moreover, each fly began as a larva that burrowed through a homogeneous artifical medium and then completed its development as a pupa 2 to 3 millimeters long attached to the surface of a glass vial. No sensible definition of environment would allow that it was different on the left and right sides, yet the two halves of the organism did indeed develop differently. This random asymmetry is a consequence of developmental noise, the random events at a cellular and molecular level that influence cell division and maturation and that especially may result in small differences in the time when critical changes occur. If a cell divides too early, it may give rise to an extra hair; if too late, it may not differentiate at all. Such random developmental events contribute a significant amount of the variation of an organism. For very complex organs like the brain, in which small structural variations may be reflected in large functional differences, random developmental noise may be an important source of individual variation.
At present the connections among gene, environment, and such traits as shape, size, and behavior are known only at a superficial, phenomenological level. The actual mechanisms of interaction are unknown, but some simple cases of control of enzyme production and activity provide a model for the more complex cases. Information from the environment plays a role at four levels. At the lowest level the synthesis of a particular enzyme or protein is turned on or off because the gene specifying that protein is either blocked or made available to the machinery of protein synthesis by the very substances on which the enzyme will operate. For example, in bacteria the gene for the enzyme that splits lactose is normally blocked, but if lactose is present in the environment, it combines in the cell with the blocking molecule and opens the gene to the protein synthetic machinery. Conversely, in the same bacteria the genes for enzymes that synthesize the essential amino acid tryptophane are normally turned on, but as tryptophane accumulates, it combines with a blocking molecule and turns the gene off. At a higher level, substances acted upon by enzymes may combine with the enzymes to stabilize them and so prevent their degradation, thus guaranteeing an adequate supply when the enzyme is in high demand. At yet a higher level, the normal kinetics of chemical reactions slow down a chain of synthetic events if the end product accumulates and speed it up if the end product is rapidly removed. At the highest level, the rate of protein synthesis in general is modulated by temperature, nutrients, and inorganic ions by changes in the rate of production of the enzymes necessary for synthesis.
Each of these mechanisms except the last has the property that information from the environment modulates the biosynthetic pathways in a way that matches the rate of activity to the demand for the product. The sensitivity of total biosynthetic activity to temperature and nutrients cannot really be regarded as adaptive, however, but is a mechanical consequence of general properties of chemical systems.
The consequence of the interaction of gene, environment, and developmental noise is a many-to-many relationship between gene and organism. The same genotype gives rise to many different organisms, and the same organism can correspond to many different genotypes. That does not mean that the organism is infinitely plastic, or that any genotype can correspond to any phenotype. Norms of reaction for different genotypes are different, but it is the norms of reaction that are the proper object of study for developmental biologists rather than some ideal organism that is supposed to be produced deterministically by the genes.
The view of development as the inevitable unfolding of successive stages, although incorrect, does incorporate an important feature of ontogeny, that it is a historical process in which the next event is influenced by the previous state. Development is then a contingent process in which the effect of a force cannot be specified in general but only in a particular context. One consequence of this contingency is that the environment as it is relevant to a developing organism is a temporal sequence of events in which the exact order is critical. If a heat shock is given to some strains of Drosophila during a critical four-hour period of their development, the pattern of veins in the wing will be altered. A shock given before or after this critical period will not change the wing veins but may affect other traits such as eye size. But this temporal contingency is also contingent on genotype, since genetically different individuals may develop identically in some environmental sequences but differ from each other in other environments. Most flies develop a normal wing pattern at 25°C, but only some genotypes produce abnormal wing veins under heat shock.
A second consequence of developmental contingency is that the effect of genetic variation on development of a trait depends upon genes other than those directly concerned with the character. The experiments of Rendel (1967) and Waddington (1957) on so-called “canalized” characters have shown that, paradoxically, traits that do not vary from individual to individual nevertheless have a genetic basis for variation. The number of saltellar bristles, four, on the back of a Drosophila is extremely constant from fly to fly. If, however, the development of the fly is severely upset experimentally, flies under the same treatment will develop different numbers of bristles. These differences turn out to be heritable, so there is indeed genetic variation among individuals that would affect bristle number if the normal developmental system did not damp out the variation. Moreover, the buffered developmental system itself turns out to be a consequence of yet other genes, so it is possible genetically to remove the damping or to alter its characteristics so that it buffers around six bristles instead of four.
Yet another consequence of developmental contingency is that ontogeny is not a linear array of stages, one leading always to a particular next stage, but a branched set of pathways. At one extreme, the leaves and stems of tropical vines take a variety of shapes and thicknesses, depending upon where the growing tip is in relation to the ground. There is one form while the vine is growing along the ground, a second when it begins to climb a tree, a third when it reaches a great height, and a fourth when it descends from the tree branches, hanging freely in the air. Any one of these forms will succeed any other, depending upon environmental cues of light, gravity, and contact, so each state is accessible from all the others, and the transition from one state to another has only a weak dependence on the previous history of growth. Such vines are at one extreme of the structure of developmental pathways in which the probability of entering any developmental sequence is essentially independent of the present state or past history. At the other extreme are unique transitions in which a given developmental step can only be taken from a particular state, and the system is irreversible. Once a developing bud is committed to floral development, the process cannot be reversed to make a leaf.
Developmental processes in general fall between these extremes, with early stages in development being both reversible and multiply branched. As development proceeds, many traits become irreversibly fixed. In Drosophila clumps of embryonic tissue normally destined to become genitalia, legs, wings, or eyes of adults, can develop into a different adult tissue if they are held long enough in an embryonic, undifferentiated state. Genital cells can change to either legs or antennae, but the reverse cannot happen. Embryonic leg and antenna cells can change reversibly to wing, and wing reversibly to eye, but embryonic eye will never change to antenna. So there is a topology of possible developmental transitions that puts constraints on developmental pathways without making them unique.
Finally, the processes of development are Markovian. That is, the probability of transition to a given state depends upon the state of the organism at the time of the transition but not on how it came into that state. Small seeds give rise, in general, to small seedlings, which grow slowly because they are shaded by competitors. It does not matter whether the seed was small because of the maternal plant’s genotype or because it set seed in an unfavorable habitat. Small animals, with large surface-to-volume ratios, lose a great deal of heat by radiation, irrespective of the causes of their small size. Thus the organism, irrespective of the internal and external forces that influenced it, enters directly into the determination of its own future. The view of development that sees genes as determinative, or even a view that admits interaction between gene and environment as determining the organism, places the organism as the end point, the object, of forces. The arrows of causation point from gene and environment to organism. In fact, however, the organism participates in its own development because the outcome of each developmental step is a precondition of the next. But the organism also actively participates in its own development because, as we shall see, it is the determinant of its own milieu.
Organism and environment
The classical Darwinian view of the process of evolution places the problem of adaptation as one of the two aspects of nature that must be understood: “In considering the Origin of Species, it is quite conceivable that a naturalist . . . might come to the conclusion that species had not been independently created, but had descended like varieties, from other species. Nevertheless, such a conclusion, even if well founded, would be unsatisfactory, until it could be shown how the innumerable species inhabiting this world have been modified, so as to acquire that perfection of structure and coadaptation which justly excites our admiration. “(Darwin 1859). Darwin’s solution, of course, was that different variants within a species possess properties that make them more or less successful in the struggle for existence. There are two ways in which this differential success can be viewed. The first, purely kinetic, view is that different variants simply have different reproductive rates and probabilities of survival, so in the end one type will come to characterize the species. Nothing in this description, however, predicts that “perfection which so excites our admiration.” One genotype having a slightly higher egg-laying rate than another at high temperatures would result in evolution but not in any impression of the marvelous fit between organism and the external world.
The second view, however, does explain the apparent fit. It is that the external world poses certain well-defined “problems” for organisms; those that best survive and reproduce are those whose morphological, physiological, and behavioral traits represent the best solutions to the problems. So locomotion is a problem that swimming animals have solved by developing flattened appendages such as fins, flukes, and webbed feet; terrestrial animals have solved it by developing hooves, paws, and articulated legs; and flying animals have solved it by developing wings. This view of adaptation acquired credibility not only from an appeal to the findings of common sense and engineering—that fins really do help movement through water, and wings through air—but also from the fact that insects, bats, and birds have all developed wings from quite different anatomical features. Such convergent homologies make it seem obvious that flying is a problem and that independent solutions have evolved through natural selection. Organisms are the objects of the force of natural selection. This force sorts out the form that is the best solution to the problem posed by the external world. The word “adaptation” reflects this point of view, implying that the organism is molded and shaped to fit into a preexistent niche, given by the autonomous forces of the environment, just as a key is cut and filed to fit into a lock.
There are two difficulties with this formulation of evolution, one conceptual and the other factual. The conceptual problem is how to define the niche of a potential organism before the organism exists. The physical world can be put together is an uncountable infinity of ways to create niches. One can construct an arbitrary number of menus of food items, say particular frequencies of different plant species which would nourish an insect, but which no insect actually eats. No animal crawls on its stomach, lays eggs, and eats grass, although snakes live in the grass. No bird eats the leaves at the tops of trees, although lots of insects do. If evolution is now going on, as we assume it is, then what marks out the combinations of physical and biotic factors that make the niches into which organisms are evolving? Is this a natural class? Could we somehow discover physical rules that would delimit the niches for us and show us that all other conceivable combinations of physical and biological factors for some reason do not constitute niches? An insight into this question can be gained by consulting the description of ecological niches in works on ecology. The description of the niche of a bird, for example, is a list of what the bird eats, of what and where it builds its nest, how much time it spends foraging in different parts of the trees or ground, what its courtship pattern is, and so on. That is, the niche is described always in terms of the life activity of the bird itself. This is not simply a convenience but an implicit recognition that niches are defined in practice by the organisms in the process of their activities. But there is a contradiction here. If the metabolism, anatomy, and behavior of an organism define its niche, how can a niche exist before the species, so that the species can evolve into it? This contradiction is not resolved in the classical Darwinian theory of adaptation, which depends absolutely on the problem preexisting the solution.
A weak claim is sometimes made that there are indeed preferred organizations of the external world, but that we simply do not know how to find them, although organisms do so in their evolution. Once again, convergence of unrelated forms is offered as evidence. The marsupial fauna of Australia has a number of forms that closely resemble placental mammals, although their evolution has been totally independent. There are marsupial “wolves,” “moles,” “rabbits,” “bears,” and “rats,” and sometimes the superficial resemblance to the placental mammal is striking, as in the case of the “rats” and “wolves.” On the other hand, there are no marsupial whales, bats, or ungulates, so niches are not inevitably filled. Nevertheless, if niches do not exist independently of organisms, some other explanation of convergence must be found.
The factual difficulty of formulating evolution as a process of adapting to preexistent problems is that the organism and the environment are not actually separately determined. The environment is not a structure imposed on living beings from the outside but is in fact a creation of those beings. The environment is not an autonomous process but a reflection of the biology of the species. Just as there is no organism without an environment, so there is no environment without an organism. The construction of environments by species has a number of well-known aspects that need to be incorporated into evolutionary theory.
Organisms determine what is relevant. The bark of trees is part of the woodpecker’s environment, but the stones at the base of the tree, even though physically present, are not. On the other hand, thrushes that break snail shells include the stones but exclude the tree from their environment. If breaking snail shells is a problem to which the use of a stone anvil is a thrush’s solution, it is because thrushes have evolved into snail-eating birds, whereas woodpeckers have not. The breaking of snail shells is a problem created by thrushes, not a transcendental problem that existed before the evolution of the Turdidae.
Not only do organisms determine their own food, but they make their own climate. It is well known in biometeorology that the temperature and moisture within a few inches of the soil in a field is different from the conditions on a forest floor or at the top of the forest canopy. Indeed, the microclimate is different on the upper and lower surfaces of a leaf. Which of these climates constitutes an insect’s environment depends upon its habitat, a matter that, in a gross sense, is coded in the insect’s genes. All terrestrial organisms are covered with a boundary layer of warm air created by the organism’s metabolism. Small ectoparasites living in that boundary layer are insulated from the temperature and moisture conditions that exist a few millimeters off the surface of their host. If the ectoparasite should evolve to become larger, it will emerge from the warm, moist boundary layer into the cold stratosphere above, creating a totally new climatic environment for itself. It is the genes of lions that make the savannah part of their environment, and the genes of sea lions that make the ocean their environment, yet lions and sea lions have a common carnivore ancestor. When did swimming, catching fish, and holding air in its lungs become problems for the terrestrial carnivore from which sea lions evolved?
Organisms alter the external world as they interact with it. Organisms are both the consumers and the producers of the resources necessary to their own continued existence. Plant roots alter the physical structure and chemical composition of the soil in which they grow, withdrawing nutrients but also conditioning the soil so that nutrients are more easily mobilized. Grazing animals actually increase the rate of production of forage, both by fertilizing the ground with their droppings and by stimulating plant growth by cropping. Organisms also influence the species composition of the plant community on which they depend. White pine trees in New England make such a dense shade that their own seedlings cannot grow up under them, so hardwoods come in to take their place. It is the destruction of the habitat by a species that leads to ecological succession. On the other hand, organisms may make an environment more hospitable for themselves, as when beavers create ponds by felling trees and building dams; indeed, a significant part of the landscape in northeastern United States has been created by beavers.
The most powerful change of environment made by organisms is the gas composition of the atmosphere. The terrestrial atmosphere, consisting of 80 percent nitrogen, 18 percent oxygen, and a trace of carbon dioxide, is chemically unstable. If it were allowed to reach an equilibrium, the oxygen and nitrogen would disappear, and the atmosphere would be nearly all carbon dioxide, as is the case for Mars and Venus. It is living organisms that have produced the oxygen by photosynthesis and that have depleted the carbon dioxide by fixing it in the form of carbonates in sedimentary rock. A present-day terrestrial species is under strong selection pressure to live in an atmosphere rich in oxygen and poor in carbon dioxide, but that metabolic problem has been posed by the activity of the living forms themselves over two billion years of evolution and is quite different from the problem faced by the earliest metabolizing cells.
Organisms transduce the physical signals that reach them from the outside world. Fluctuations in temperature reach the inner organs of a mammal as chemical signals, not thermal signals. The regulatory system in mammals alters the concentration of sugar and various hormones in the blood in response to temperature. Ants that forage only in the shade detect temperature changes as such only momentarily, but over a longer term will experience sunshine as hunger. When a mammal sees and hears a rattlesnake, the photon energy and vibrational energy that fall on its eyes and ears are immediately transformed by the neurosecretory system into chemical signals of fear. On the other hand, another rattlesnake will react very differently. It is the biology of each species that determines what physical transformation will occur when physical signals impinge on the organism or whether these signals are even perceived. Bees can see light in the ultraviolet range, but mammals cannot. For bees, ultraviolet light leads to a source of food, while for us it leads to skin cancer. One of the most striking aspects of evolution is the way in which the significance of physical signals has been completely altered in the origin of new species.
Organisms transform the statistical pattern of environmental variation in the external world. Both the amplitude and the frequency of external fluctuations are transformed by biological processes in the organism. Fluctuations are damped by various storage devices that average over space and time. An animal with a wide home range averages food availability over smaller spatial patches. Fat or carbohydrate storage averages the fluctuating availability of resources in time. All seeds store solar energy during the growing season in order to provide it to seedlings, which are at first unable to photosynthesize, Animals in turn store the seeds and thus capture the plant storage mechanism, while converting the storage cycle to their own biological rhythms. Human beings have added yet a third form of damping by engaging in planned production that responds to fluctuations in demand,
Conversely, organisms can magnify small fluctuations, as when birds use a small change in the abundance of a food item as a signal to shift their search images to another item. Living beings can also integrate and differentiate signals. Plants flower when a sufficient number of degree-days above a critical temperature have been accumulated, irrespective of the detailed day-to-day fluctuations in temperature. On the other hand, Cladocera change from asexual to sexual reproduction in response to a rapid change in temperature, food availability, or oxygen concentration, irrespective of the actual level itself. An animal’s visual acuity depends upon the rate of change of light intensity at the edges of objects, rather than on the total intensity itself. The frequency of external oscillations can even be converted to a cycle having a different frequency. The thirteen- or seventeen-year periodic cicadas hatch out after thirteen or seventeen successive seasonal cycles in the temperate zone, so somehow they are able to count up to a prime number.
The organism-environment relationship defines the “traits” selected. Suppose, for example, that a lizard lives in an equable climate in which food is abundant but must be caught by stalking and pouncing. Since the lizard must expend energy carrying its whole weight as it hunts—and its effectiveness in catching prey may depend on its size—the size spectrum of insect prey may be a major selective force acting on lizard size, while the spatial distribution of prey may determine the lizard’s preferences for certain locations over others. The size and preference together form a trait, “predation effectiveness.” Now if the climate becomes hotter, the lizard faces a physiological problem, the danger of overheating. Since the rate of heating is affected by body color and the surface- volume relation, body size and color are now linked as part of the physiological trait “heat tolerance.” Genes affecting color and size will show epistatic interaction in their effect on this trait, even if the biochemical products of these genes’ activity never meet and even if temperature does not affect growth rate. The course of selection, the degree of change in size against that in color, will depend on the available genetic variance for color and size, the other selection forces operating on both of these, and the intensity or frequency of heat stress. This last factor depends on exposure, where the lizard spends its time. Its preference for certain locations becomes part of its ecological heat tolerance, which includes physiological tolerance and exposure. So now location preference, which may have evolved in relation to prey habitat selection, and body size, related to prey size, become linked together with color in “heat tolerance” and continue to be linked in the trait “predation effectiveness.”
Suppose now that a predator enters the scene. The lizard may avoid the predator by camouflaging itself or by changing its haunts. Now color and site selection have become linked as part of the trait “predator avoidance,” while still forming part of “heat tolerance.” Furthermore, a change in where the lizard spends its time can either intensify or diminish selection for “heat tolerance” and, by changing the color of the substrate where it is found, alter the camouflage significance of body color and therefore its effectiveness in heat tolerance. If a second lizard species is present, feeding on the same array of insects, then size, location, and possibly heat tolerance become part of the new trait “competitive ability.”
Thus, under natural conditions, aspects of phenotype are constantly joining together and coming apart to create and destroy “traits,” which are then selected. The opposite side of organisms constructing their environment is that the environment constructs the traits by means of which the organisms solve the problems posed to them by the environments they created.
Of course, under conditions of artificial selection, the selectors define the traits. Any arbitrary combination of measurements may be defined as a trait. If the price of soybean cake is favorable, the dry weight of soybeans may be the defined “yield” and thus be a trait for selection. With a change in the market, “yield” may become oil per hectare. Or an experimenter may find that some laboratory rats, when picked up by their tails, bite the technician. The experimenter might define the frequency of biting the technician as “aggressivity” and report that he has selected for increased or diminished “aggression” in rats, even if the causal pathway is that the rats with more sensitive tails bite more.
Therefore, when we talk about the traits of organisms fitting their environments, we have to remember that neither trait nor environment exists independently. Nothing better illustrates the error of the problemsolution model than the seemingly straightforward example of the horse’s hoof given by Lorenz (1962). The “central nervous apparatus does not prescribe the laws of nature any more than the hoof of the horse prescribes the form of the ground . . . But just as the hoof of the horse is adapted to the ground of the steppe which it copes with, so our central nervous apparatus for organizing the image of the world is adapted to the real world with which man has to cope . . . The hoof of the horse is already adapted to the ground of the steppe before the horse is born and the fin of the fish is adapted to the water before the fish hatches. No sensible person believes that in any of these cases the form of the organ ‘prescribes’ its properties to the subject,”
Indeed, there is a real world out there, but Lorenz makes the same mistake as Ruskin, who believed in the “innocent eye.” It is a long way from the “laws of nature” to the horse’s hoof. Rabbits, kangaroos, snakes, and grasshoppers, all of whom traverse the same ground as the horse, do not have hooves. Hooves come not from the nature of the ground but from an animal of certain size, with four legs, running, not hopping, over the ground at a certain speed and for certain periods of time. The small gracile ancestors of the horse had toes and toenails, not hooves, and they got along very well indeed. So, too, our central nervous systems are not fitted to some absolute laws of nature, but to laws of nature operating within a framework created by our own sensuous activity. Our nervous system does not allow us to see the ultraviolet reflections from flowers, but a bee’s central nervous system does. And bats “see” what nighthawks do not. We do not further our understanding of evolution by general appeals to “laws of nature” to which all life must bend. Rather we must ask how, within the general constraints of the laws of nature, organisms have constructed environments that are the conditions for their further evolution and reconstruction of nature into new environments.
It is difficult to think of any physical force or universal physical law that represents a fixed problem to which all organisms must find a direct solution. We think of gravitation as universal, but because it is such a weak force, it does not apply in practice to very small organisms suspended in liquid media. Bacteria are largely outside the influence of gravity as a consequence of their size, that is, as a consequence of their genes. On the other hand, they are subject to another universal physical force, Brownian motion of molecules, which we are protected from by our large size, again a result of our evolution. The most remarkable property of living organisms is that they have avoided biologically the chemical laws of mass action and the high energy needed to initiate most chemical reactions; both have been accomplished by structure. The structure of the genes themselves, and the way they are held together in very large macromolecular structures, makes it possible for gene replication and protein synthesis to take place even though there is only a single molecule of each gene in each cell. The structure of enzymes, in turn, makes it possible to carry out at ambient temperatures chemical reactions that would otherwise require great heat.
It is impossible to avoid the conclusion that organisms construct every aspect of their environment themselves. They are not the passive objects of external forces, but the creators and modulators of these forces. The metaphor of adaptation must therefore be replaced by one of construction, a metaphor that has implications for the form of evolutionary theory. With the view that the organism is a passive object of autonomous forces, evolutionary change can be represented as two simultaneous differential equation systems. The first describes the way in which organism O evolves in response to environment E, taking into account that different species respond to environments in different ways:

The second is the law of autonomous change of the environment as some function only of environmental variables:

A constructionist view that breaks down the alienation between the objectorganism and the subject-environment must be written as a pair of coupled differential equations in which there is coevolution of the organismenvironment pair:
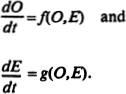
There is already a parallel for such a coevolutionary system in the theory of the coevolution of prey and predator or host and parasite. The prey is the environment of the predator, and the predator the environment of the prey. The coupled differential equations that describe their coevolution are not easy to solve, but they represent the minimum structure of a correct theory of the evolution of such systems. It is not only that they are difficult to solve, but that they pose a conceptual complication, for there is no longer a neat separation between cause (the environment) and effect (the organism). There is, rather, a continuous process in which an organism evolves to solve an instantaneous problem that was set by the organism itself, and in evolving changes the problem slightly. To understand the evolution of the sea lion from a primitive carnivore ancestor, we must suppose that at first the water was only a marginal habitat putting only marginal demands on the animal. A slight evolution of the animal to meet these demands made the aquatic environment a more significant part of the energetic expenditure of the proto-sea lion, so a shift in selective forces operated instantaneously on the shape of its limbs. Each change in the animal made the environment more aquatic, and each induced change in the environment led to further evolution of the animal.
The incorporation of the organism as an active subject in its own ontogeny and in the construction of its own environment leads to a complex dialectical relationship of the elements in the triad of gene, environment, and organism. We have seen that the organism enters directly and actively by being an influence on its own further ontogeny. It enters by a second indirect pathway through the environment in its own ontogeny. The organism is, in part, made by the interaction of the genes and the environment, but the organism makes its environment and so again participates in its own construction. Finally, the organism, as it develops, constructs an environment that is a condition of its survival and reproduction, setting the conditions of natural selection. So the organism influences its own evolution, by being both the object of natural selection and the creator of the conditions of that selection. Darwin’s separation of ontogeny and phylogeny was an absolutely necessary step in shaking free of the Lamarckian transformationist model of evolution. Only by alienating organism from environment and rigorously separating the ontogenetic sources of variation among organisms from the phylogenetic forces of natural selection could Darwin put evolutionary biology on the right track. So, too, Newton had to separate the forces acting on bodies from the properties of the bodies themselves: their mass and composition. Yet mass and energy had to be reintegrated to resolve the contradictions of the strict Newtonian view and to make it possible for modern alchemy to turn one element into another. In like manner, Darwinism cannot be carried to completion unless the organism is reintegrated with the inner and outer forces, of which it is both the subject and the object.
TWO On Analysis
4 The Analysis of Variance and the Analysis of Causes
This chapter was first published in American Journal of Human Genetics 26 (1974): 400-411.
TWO ARTICLES by Newton Morton (1974) and his colleagues (Rao, Morton, and Yee 1974) provide a detailed analytic critique of various estimates of heritability and components of variance for human phenotypes. They make especially illuminating remarks on the problems of partitioning variances and covariances among groups such as social classes and races. The most important point of all, at least from the standpoint of the practical, social, and political applications of human population genetics, occurs at the conclusion of the first paper, in which Morton points out explicitly the chief programmatic fallacy committed by those who argue so strongly for the importance of heritability measures for human traits. The fallacy is that a knowledge of the heritability of some trait in a population provides an index of the efficacy of environmental or clinical intervention in altering the trait either in individuals or in the population as a whole. This fallacy, sometimes propagated even by geneticists, who should know better, arises from the confusion between the technical meaning of heritability and the everyday meaning of the word. A trait can have a heritability of 1.0 in a population at some time, yet this could be completely altered in the future by a simple environmental change. If this were not the case, “inborn errors of metabolism” would be forever incurable, which is patently untrue. But the misunderstanding about the relationship between heritability and phenotypic plasticity is not simply the result of an ignorance of genetics on the part of psychologists and electronic engineers. It arises from the entire system of analysis of causes through linear models, embodied in the analysis of variance and covariance and in path analysis. It is indeed ironic that while Morton and his colleagues dispute the erroneous programmatic conclusions that are drawn from the analysis of human phenotypic variation, they nevertheless rely heavily for their analytic techniques on the very linear models that are responsible for the confusion.
We would like to look rather closely at the problem of the analysis of causes in human genetics and to try to understand how the underlying model of this analysis molds our view of the real world. We will begin by saying some very obvious and elementary things about causes, but we will come thereby to some very annoying conclusions.
Discrimination of causes and analysis of causes
We must first separate two quite distinct problems about causation that Morton discusses. One is to discriminate which of two alternative and mutually exclusive causes lies at the basis of some observed phenotype. In particular, it is the purpose of segregation analysis to attempt to distinguish those individuals who owe their phenotypic deviation to their homozygosity for rare deleterious gene alleles from those whose phenotypic peculiarity arises from the interaction of environment with genotypes drawn from the normal array of segregating genes of minor effect. This is the old problem of distinguishing major gene effects from “polygenic” effects. We do not want to take up here the question of whether such a clear distinction can be made or whether the spectrum of gene effects and gene frequencies is such that we cannot find a clear dividing line between the two cases. The evidence at present is ambiguous, but at least in principle it may be possible to discriminate two etiologic groups, and whether such groups exist for any particular human disorder is a matter for empirical research. It is possible, although not necessary, that the form of clinical or environmental intervention required to correct a disorder arising from homozygosity for a single rare recessive allele (the classical “inborn error of metabolism”) may be different from that required for the “polygenic” class. Moreover, for the purposes of genetic counseling, the risk of future offspring being affected will be different if a family is segregating for a rare recessive than if it is not. Thus the discrimination between two alternative causes of a human disorder is worth making if it can be done.
The second problem of causation is quite different. It is the problem of the analysis into separate elements of a number of causes that are interacting to produce a single result. In particular, it is the problem of analyzing into separate components the interaction between environment and genotype in the determination of phenotype. Here, far from trying to discriminate individuals into two distinct and mutually exclusive etiologic groups, we recognize that all individuals owe their phenotype to the biochemical activity of their genes in a unique sequence of environments and to developmental events that may occur subsequent to, although dependent upon, the initial action of the genes. The analysis of interacting causes is fundamentally a different concept from the discrimination of alternative causes. The difficulties in the early history of genetics embodied in the pseudoquestion of “nature versus nurture” arose precisely because of the confusion between these two problems in causation. It was supposed that the phenotype of an individual could be the result of either environment or genotype, whereas we understand the phenotype to be the result of both. This confusion has persisted into modern genetics with the concept of the phenocopy, which is supposed to be an environmentally caused phenotypic deviation, as opposed to a mutant which is genetically caused. But, of course, both “mutant” and “phenocopy” result from a unique interaction of gene and environment. If they are etiologically separable, it is not by a line that separates environmental from genetic causation but by a line that separates two kinds of genetic basis: a single gene with major effect or many genes each with small effect. That is the message of the work by Waddington (1953) and Rendel (1959) on canalization.
Quantitative analysis of causes
If an event results from the joint operation of a number of causative chains, and if these causes “interact” in any generally accepted meaning of the word, it becomes conceptually impossible to assign quantitative values to the causes of that individual event. Only if the causes are utterly independent could we do so. For example, if two men lay bricks to build a wall, we may quite fairly measure their contributions by counting the number laid by each; but if one mixes the mortar and the other lays the bricks, it would be absurd to measure their relative quantitative contributions by measuring the volumes of bricks and of mortar. It is obviously even more absurd to say what proportion of a plant’s height is owed to the fertilizer it received and what proportion to the water, or to ascribe so many inches of a man’s height to his genes and so many to his environment. But this obvious absurdity appears to frustrate the universally acknowledged program of Cartesian science to analyze the complex world of appearances into an articulation of causal mechanisms. In the case of genetics, it appears to prevent our asking about the relative importance of genes and environment in the determination of phenotype. The solution offered to this dilemma, a solution that has been accepted in a great variety of natural and social scientific practice, has been the analysis of variation. That is, if we cannot ask how much of an individual’s height is the result of its genes and how much a result of its environment, we will ask what proportion of the deviation of height from the population mean can be ascribed to deviation of environment from the average environment and how much to the deviation of this genetic value from the mean genetic value. This is the famous linear model of the analysis of variance, which can be written as

where μY is the mean score of all individuals in the population; Y is the score of the individual in question; G is the average score of all individuals with the same genotype as the one in question; E is the average score of all individuals with the same environment as the one in question; GE, the genotype-environment interaction, is that part of the average deviation of individuals sharing the same environment and genotype that cannot be ascribed to the simple sum of the separate environmental and genotypic deviations; and e takes into account any individual deviation not already consciously accounted for and assumed to be random over all individuals (measurement error, developmental noise, and so on).
We have written this well-known linear model in a slightly different way than it is usually displayed in order to emphasize two of its properties that are well known to statisticians. First, the environmental and genotypic effects are in units of phenotype. We are not actually assessing how much variation in environment or genotype exists, but only how much perturbation of phenotype has been the outcome of average difference in environment. The analysis in eq. (1) is completely tautological, since it is framed entirely in terms of phenotype, and both sides of the equation must balance by the definitions of GE and e. To turn expression (1) into a contingent one relating actual values of environmental variables, such as temperature, to phenotypic score, we would need functions of the form:

and

where g and T are measured on a genetic and a temperature scale rather than on a scale of phenotype. Thus the linear model, eq. (1), makes it impossible to know whether the environmental deviation (E-μY) is small because there are no variations in actual environment or because the particular genotype is insensitive to the environmental deviations, which may be quite considerable. From the standpoint of the tautological analysis of eq. (1), this distinction is irrelevant, but as we shall see, it is supremely relevant for those questions that are of real importance in our science.
Second, eq. (1) contains population means at two levels. One level is the grand mean phenotype μY, and the other is the set of so-called marginal genotypic and environmental means, E and G. These, it must be remembered, are the mean for a given environment averaged over all genotypes in the population and the mean for a given genotype averaged over all environments.
But since the analysis is a function of these phenotypic means, it will, in general, give a different result if the means are different. That is, the linear model is a local analysis. It gives a result that depends upon the actual distribution of genotypes and environments in the particular population sampled. Therefore, the result of the analysis has a historical (spatiotemporal) limitation and is not a general statement about functional relations. So the genetic variance for a character in a population may be very small because the functional relationship between gene action and the character is weak for any conceivable genotype, or it may be small simply because the population is homozygous for those loci that are of strong functional significance for the trait. The analysis of variation cannot distinguish between these alternatives, even though for most purposes in human genetics we wish to do so.
What has happened in attempting to solve the problem of the analysis of causes by using the analysis of variation is that a totally different object has been substituted as the object of investigation, almost without our noticing it. The new object of study, the deviation of phenotypic value from the mean, is not the same as the phenotypic value itself, and the tautological analysis of that deviation is not the same as the analysis of causes. In fact, the analysis of variation throws out the baby with the bath water. It is both too specific in that it is spatiotemporally restricted in its outcome and too general in that it confounds different causative schemes in the same outcome. Only in a very special case, to which we shall refer below, can the analysis of variation be placed in a one-to-one correspondence to the analysis of causes.
Norm of reaction
The real object of study, both for programmatic and theoretical purposes, is the relation among genotype, environment, and phenotype. This is expressed in the norm of reaction, which is a table of correspondence between phenotype, on the one hand, and genotype-environment combinations on the other. The relations between phenotype and genotype and between phenotype and environment are many-many relations, no single phenotype corresponding to a unique genotype and vice versa.
In order to clarify the relation between the two objects of study (that is, the norm of reaction and the analysis of variance, which analyzes something quite different), let us consider the simplified norms of reaction shown in Fig. 4.1 a-h. We assume that there is a single well-ordered environmental variable E, say temperature, and a scale of phenotypic measurement P. Each line is the norm of reaction, the relationship of phenotype to environment, for a particular hypothetical genotype (G 1 or G 2).
The first thing to observe is that in every case the phenotype is sensitive to differences in both environment and genotype. That is, each genotype reacts to changing environment, and in no case are the two genotypes identical in their reactions. Thus in any usual sense of the word, both genotypes and environment are causes of phenotypic differences and are necessary objects of our study.
Figure 4.1 a is in one sense the most general, for if environment extends uniformly over the entire range and if the two genotypes are equally frequent, there is an overall effect of genotype (G 1 being on the average superior to G2) and an overall effect of environment (phenotype gets smaller on the average with increasing temperature). Nevertheless, the genotypes cross, so neither is always superior.
Figure 4.1 b shows an overall effect of environment, since both genotypes have a positive slope, but there is no overall effect of genotype, since the two genotypes would have exactly the same mean phenotype if all environments were considered equally. There is no a priori way from Fig. 4.1 b of ranking the two genotypes. However, if because of particular circumstances the distribution of environments were heavily weighted toward the lower temperatures, then G 1 would be consistently superior to G2; an analysis of variance would show a strong effect of genotype as well as of environment, but very little genotype-environment interaction. Thus the analysis of variance would reflect the particular environmental circumstances and give a completely incorrect picture of the general relationship between cause and effect here, where there is overall no effect of genotype but a strong genotype-environment interaction.
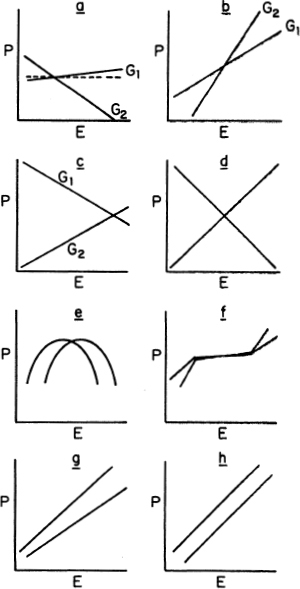
Fig. 4.1. Examples of different forms of reaction norms. In each case the phenotype (P) is plotted as a function of environment (E) for different genotypes (G1, G 2).
Figure 4.1 c is the complementary case to that shown in 4.1 b. In 4.1 c there is no overall effect of environment, but G 1 is clearly superior to G2 overall. In this case a strong environmental component of variance will appear, however, if either one of the genotypes should predominate in the population. So the historical events that mold the genotypic distribution of a population will have an effect on the judgment, from the analysis of variance, of the importance of environment.
The overall lack of genetic effect in 4.1 b and of environmental effect in 4.1 c can both appear in a trait like that shown in 4.1a, which overall has both effects if the distribution of environments or of genotypes is asymmetric. Thus if environments are distributed around the middle in 4.1a, there will appear to be no average effect of genotype, while if the population is appropriately weighted toward an excess of G 1, the average phenotype across environments will be constant, as shown by the dashed line. Here real overall effects are obscured because of spatiotemporal events, and the analysis of variance fails to reveal significant overall differences.
These last considerations lead to two extremely important points about the analysis of variance. First, although eq_(1), appears to isolate distinct causes of variation into separate elements, it does not do so, because the amount of environmental variance that appears depends upon the genotypic distribution, while the amount of genetic variance depends upon the environmental distribution. Thus the appearance of the separation of causes is a pure illusion. Second, because the linear model appears as a sum of variation from different causes, it is sometimes erroneously supposed that removing one of the sources of variation will reduce the total variance. So, the meaning of the genetic variance is sometimes given as “the amount of variation that would be left if the environment were held constant,” and the environmental variance is described as “the amount of variance that would remain if all the genetic variation were removed,” an erroneous explanation offered by Jensen (1969), for example. Suppose that the norms of reaction were as in Fig. 4.1 a and a unimodal distribution of environments were centered near the middle, with a roughly equal mixture of the two genotypes. Now suppose we fix the environment. What will happen to the total variance? That depends on which environment we fix upon. If we choose an environment about 1 SD or more to the right of the mean, there would actually be an increase in the total variance, because the difference
between genotypes is much greater in that environment than on the average over the original distribution. Conversely, suppose we fix the genotype. If we chose G2 to be our pure strain, then, again, we would increase the total variance because we had chosen the more environmentally plastic genotype. The apparent absurdity that removing a source of variance actually increases the total variance is a consequence of the fact that the linear model does not really effect a separation of causes of variation and that it is a purely local description with no predictive reliability. Without knowing the norms of reaction, the present distribution of environments, and the present distribution of genotypes, and without then specifying which environments and which genotypes are to be eliminated or fixed, it is impossible to predict whether the total variation would be increased, decreased, or remain unchanged by environmental or genetic changes.
In Fig. 4.1 d there is no overall effect of either genotype or environment, but both can obviously appear in a particular population in a particular environmental range, as discussed above.
The case shown in Fig. 4.1 e has been chosen to illustrate a common situation for enzyme activity, a parabolic relation between phenotype and environment. Here genotypes are displaced horizontally (have different temperature optima). Neither genotype is superior overall, nor is there any general monotone environmental trend for either genotype. But for any distribution of environments except a perfectly symmetrical one, there will appear a component of variance for genotypic effect. Moreover, if the temperature distribution is largely to either side of the crossover point between these two genotypes, there will be very large components of variance for both genotype and environment and a vanishingly small interaction component; yet over the total range of environments exactly the opposite is true!
Figure 4.1 e also shows a second important phenomenon, that of differential phenotypic sensitivity in different environmental ranges. At intermediate temperatures there is less difference between genotypes and less difference between the effect of environments than at more extreme temperatures. This phenomenon, canalization, is more generally visualized in Fig. 4.1 f. Over a range of intermediate phenotypes there is little effect of either genotype or environment, while outside this zone of canalization, phenotype is sensitive to both (Rendel 1959). The zone of canalization corresponds to the range of environments that have been historically the most common in the species, but in new environments much greater variance appears. Figure 4.1 f bears directly on the characteristic of the analysis of variance that all effects are measured in phenotypic units. The transformations, eqs._(2)l and (3), that express the relationship between the phenotypic deviations ascribable to genotype or environment and the actual values of the genotypes or environmental variables are not simple linear proportionalities. The sensitivity of phenotype to both environment and genotype is a function of the particular range of environments and genotypes. For the programmatic purposes of human genetics, one needs to know more than the components of variation in the historical range of environments.
Figure 4.1 a-f is meant to illustrate how the analysis of variance will give a completely erroneous picture of the causative relations among genotype, environment, and phenotype because the particular distribution of genotypes and environments in a given population at a given time picks out relations from the array of reaction norms that are necessarily atypical of the entire spectrum of causative relations. Of course it may be objected that any sample from nature can never give exactly the same result as examining the universe. But such an objection misses the point. In normal sampling procedures, we take care to get a representative or unbiased sample of the universe of interest and to use unbiased sample estimates of the parameters we care about. But there is no question of sampling here, and the relation of sample to universe in statistical procedures is not the same as the relation of variation in spatio-temporally defined populations to causal and functional variation summed up in the norm of reaction. The relative sizes of genotypic and environmental components of variance estimated in any natural population reflect in a complex way four underlying relationships: (1) the actual functional relations embodied in the norm of reaction; (2) the actual distribution of genotype frequencies—a product of long-time historical forces like natural selection, mutation, migration, and breeding structure—which changes over periods much longer than a generation; (3) the actual structure of the environments in which the population finds itself, a structure that may change very rapidly indeed, especially for human populations; and (4) any differences among genotypes that may cause a biased distribution of genotypes among environments. These differences may be behavioral (for instance, a heat-sensitive genotype may seek cooler habitats), or it may result from other individuals using the genotype as an indicator for differential treatment, since that treatment is part of environment. A causal pathway may go from tryptophane metabolism to melanin deposition to skin color to hiring discrimination to lower income, but eqJ1) would simply indicate heritability for “economic success.” The effects of historical forces and immediate environment are inextricably bound up in the outcome of variance analysis, which thus is not a tool for the elucidation of functional biological relations.
Effect of additivity
There is one circumstance in which the analysis of variance can estimate functional relationships. This is illustrated exactly in Fig. 4.1 h and approximately in 4.1 g. In these cases there is perfect or nearly perfect additivity between genotypic and environmental effects so that the differences among genotypes are the same in all environments and the differences among environments are the same for all genotypes. Then the historical and immediate circumstances that alter genotypic and environmental distributions are irrelevant. It is not surprising that the assumption of additivity is so often made, since this assumption is necessary to make the analysis of variance anything more than a local description.
The assumption of additivity is imported into analyses by four routes. First, it is thought that in the absence of any evidence, additivity is a priori the simplest hypothesis, and additive models are dictated by Occam’s razor. The argument comes from a general Cartesian world view that things can be broken down into parts without losing any essential information, and that in any complex interaction of causes, main effects will almost always explain most of what we see, while interactions will tend to be of a smaller order of importance. But this is a pure a priori prejudice. Dynamic systems in an early stage in their evolution will show rather large main effects of the forces acting to drive them, but as they approach equilibrium the main effects disappear and interactions predominate. That is what happens to additive genetic variance under selection. Exactly how such considerations apply to genotype and environment is not clear.
Second, it is suggested that additivity is a first approximation to a complex situation, and the results obtained with an additive scheme are then a first approximation to the truth. This argument is made by analogy with the expansion of mathematical functions by Taylor’s series. But this argument is self-defeating since the justification for expanding a complex system in a power series and considering only the first-order terms is precisely that one is interested in the behavior of the system in the neighborhood of the point of expansion. Such an analysis is a local analysis only, and the analysis of variance is an analysis in the neighborhood of the population mean only. By justifying additivity on this ground, the whole issue of the global application of the result is sidestepped.
Third, it is argued that if an analysis of variance is carried out and the genotype-environment interaction turns out to be small, the assumption of additivity is justified. As in the second argument, there is some circularity. As the discussion of the previous section showed, the usual outcome of an analysis of variance in a particular population in a restricted range of environments is to underestimate severely the amount of interaction between the factors that occur over the whole range.
Finally, additivity or near additivity may be assumed without offering any justification, because it suits a predetermined end. Such is the source of Fig. 4.1 g.. It is the hypothetical norm of reaction for IQ taken from Jensen (1969). It purports to show the relation between environmental “richness” and IQ for different genotypes. While there is not a scintilla of evidence to support such a picture, it has the convenient properties that superior and inferior genotypes in one environment maintain that relation in all environments, and that as environment is “enriched,” the genetic variance (and therefore the heritability) increases. This is meant to take care of those foolish egalitarians who think that spending money and energy on schools generally will iron out the inequalities in society.
Evidence on actual norms of reaction is very hard to come by. In man, measurements of reaction norms for complex traits are impossible because the same genotype cannot be tested in a variety of environments. Even in experimental animals and plants where genotypes can be replicated by inbreeding experiments or cloning, very little work has been done to characterize these norms for the genotypes that occur in natural populations and for traits of consequence to the species. The classic work of Clausen, Keck, and Heisey (1940) on ecotypes of plants shows very considerable nonadditivity of the types illustrated in Fig. 4.1 a-d.
As an example of what has been done in animals, Fig. 4.2 has been drawn from the data of Dobzhansky and Spassky (1944) on larval viability in Drosophila pseudoobscura. Each line is the reaction norm for larval viability at three different temperatures for a fourth-chromosome homozygote, where the chromosomes have been sampled from a natural population. As the figure shows, a few genotypes are of uniformly poor viability, probably corresponding to homozygosity for a single deleterious gene of strong effect. However, most genotypes are variable in their expression, and there is a great deal of genotype-environment interaction, with curves crossing each other and having quite different environmental sensitivities.